An official website of the United States government
Official websites use .gov A .gov website belongs to an official government organization in the United States.
Secure .gov websites use HTTPS A lock ( Lock Locked padlock icon ) or https:// means you've safely connected to the .gov website. Share sensitive information only on official, secure websites.
- Publications
- Account settings
- Advanced Search
- Journal List


The role of biofuels for sustainable MicrogridsF: A path towards carbon neutrality and the green economy
Mohammadali kiehbadroudinezhad, adel merabet, chaouki ghenai, ahmed g abo-khalil, tareq salameh.
- Author information
- Article notes
- Copyright and License information
Corresponding author. [email protected]
Received 2022 Oct 17; Revised 2023 Jan 27; Accepted 2023 Jan 30; Collection date 2023 Feb.
This is an open access article under the CC BY-NC-ND license (http://creativecommons.org/licenses/by-nc-nd/4.0/).
Today, with the progress of technology, the world is facing an increasing growth in power consumption. Since the fuel of most power plants is supplied from fossil fuels, it has caused an increase in global fossil fuel consumption and environmental degradation. ّFurthermore, the volatility of fossil fuel prices and unstable energy security have prompted international organizations and governments to apply policies to restrict fossil fuel use and examine alternatives to fossil fuels. Since biofuels come from renewable sources and are clean fuels, they can be an appropriate alternative to fossil fuels and play a more expansive role in supplying energy for transportation industries, power plants, and heat production systems. Although there is some research about the drawbacks of using fossil fuels and the commendation of using biofuels in various industries such as transportation, the literature lacks a comprehensive study on the evaluation and analysis of the potential of using biofuels instead of conventional fuels in power generation systems. The primary purpose of this study is to evaluate the impact of utilizing biofuels instead of fossil fuels in microgrids to achieve carbon neutrality objectives. Furthermore, this paper reviews previous research studies that have operated biofuels in three categories: solid, liquid, and gas, to generate electricity and analyzes the potential of different biofuels to produce heat and electricity for microgrid power systems. In addition to outlining the present knowledge gaps in this area, this study explores the prospects and threats associated with expanding the use of biofuels in the power production industry and the development of sustainable microgrids. This study indicated that if the technical and economic problems of employing biofuels are overcome, these clean fuels have a great potential to obtain the maximum share of the global power generation market and move toward Net Zero Emissions by 2050 Scenario (NZE) goals.
Keywords: Biofuel, Microgrids, Environment impacts, Sustainability, Power generation, Carbon neutrality, Green economy
Graphical abstract
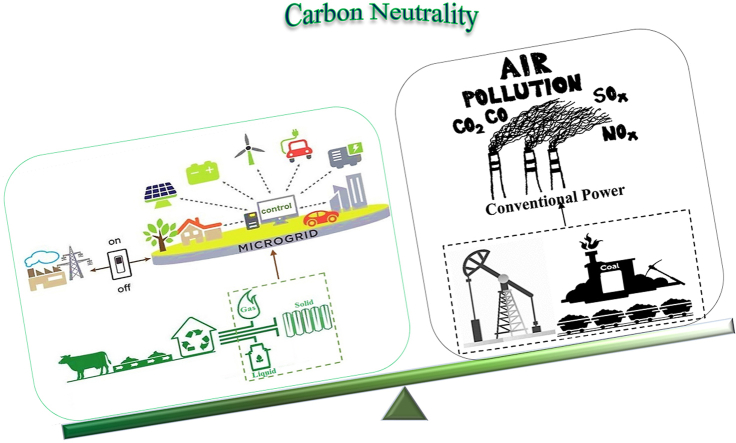
The environmental hazards of conventional power generation were studied.
Fossil fuel alternatives in power and heat generation plants were discussed.
The potential of using biofuel in future microgrids was evaluated.
Biofuel challenges and opportunities in the microgrid industry were investigated.
The application of biofuels in Islanded and on-grid microgrids was highlighted.
1. Introduction
The increasing progress of various industries and the increase of the world population in recent decades have caused a tremendous growth in power demand [ 1 ]. Environment and energy scientists expect that with the continuation of the upward trend in demand for energy in the world, the emission of greenhouse gases such as carbon dioxide will reach a new record. Also, carbon dioxide emissions are expected to increase to 37 gigatons in 2035 [ 2 ]. This issue caused crucial concerns about human health and the environment. In this context, the 1.5 °C scenario of the Paris Agreement requires governments to adopt comprehensive climate action to limit global warming by reducing greenhouse gas emissions. The world's power plants mainly rely on fossil fuels to generate power. Fossil energy sources, such as crude oil, diesel, and natural gas, cannot be reliable sources to meet the increasing electricity demands for future generations due to their exhaustible nature. Conventional energy sources also cause the emission of greenhouse gases, resulting in climate change and global warming. Due to these facts, researchers and environmental experts consider fossil fuels the most significant factor in environmental and atmospheric damage [ 3 ]. Consequently, scientists are striving to change the current power generation systems that rely on conventional energy sources to green microgrids based on clean sources. For this reason, the tendency to use renewable resources to generate power has grown dramatically in recent decades, and new and modern technologies have emerged to exploit renewable resources [ 4 ]. Fig. 1 a illustrates the growing energy consumption trend. As it is clear, according to the forecasts, the increase in energy consumption will continue until 2050, which can be a warning of the rise in greenhouse gas emissions in the future. However, according to Fig. 1 b, using renewable resources to supply the global power required will also increase, which means that renewable sources have a significant share in providing the energy needed by the world.
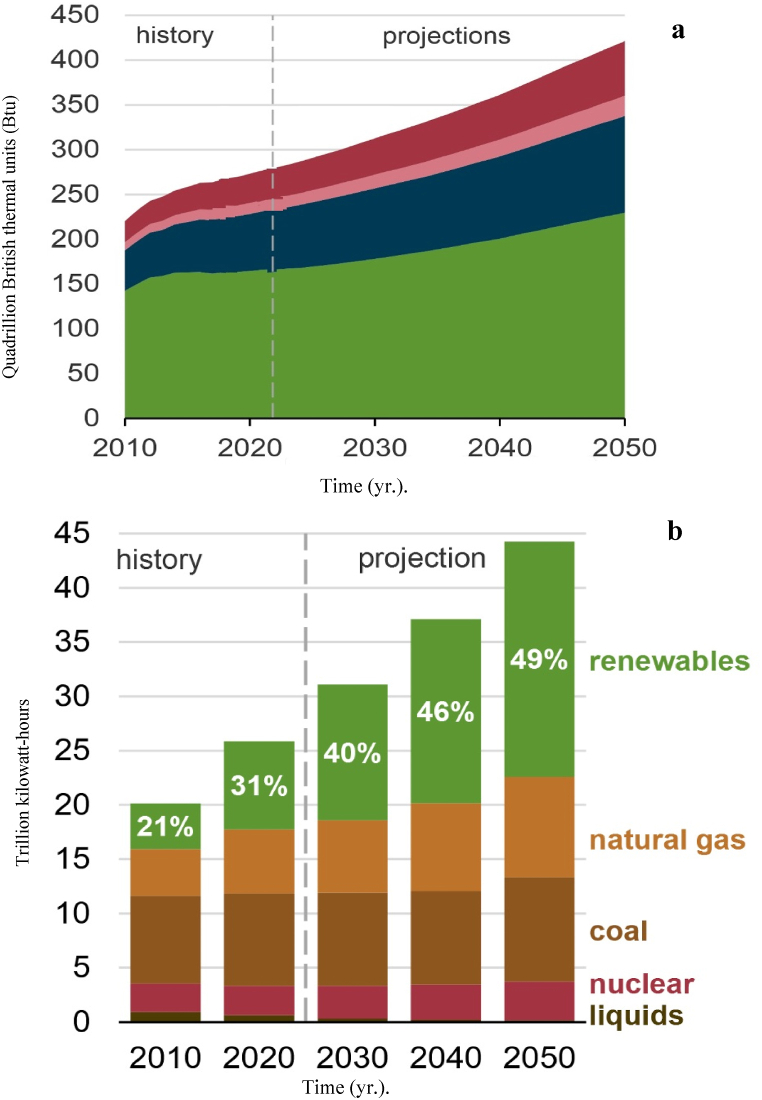
a) Global energy consumption [ 5 ], b) Global energy supply by renewable and non-renewable sources [ 5 ].
The high costs of developing conventional power plants (especially in remote areas), the emission of polluting gases, the environmental impacts, and the exhaustibility of fossil fuel reserves are the prominent factors that induced energy experts to not visualize an explicit future for the traditional power plant industry [ 6 ]. Energy and power experts believe that green microgrids, distributed generation, and energy storage systems will play a significant role in meeting the world's demand for energy, given the current power supply infrastructure and ongoing projects. As shown in Fig. 2 a, based on the statistical survey results, it is predicted that distributed generation and green microgrids will face a growth of 500% by 2027, which is a significant growth [ 7 ]. Accordingly, energy policymakers and governments excessively desire to use renewable resources as a clean and accessible source for power generation.
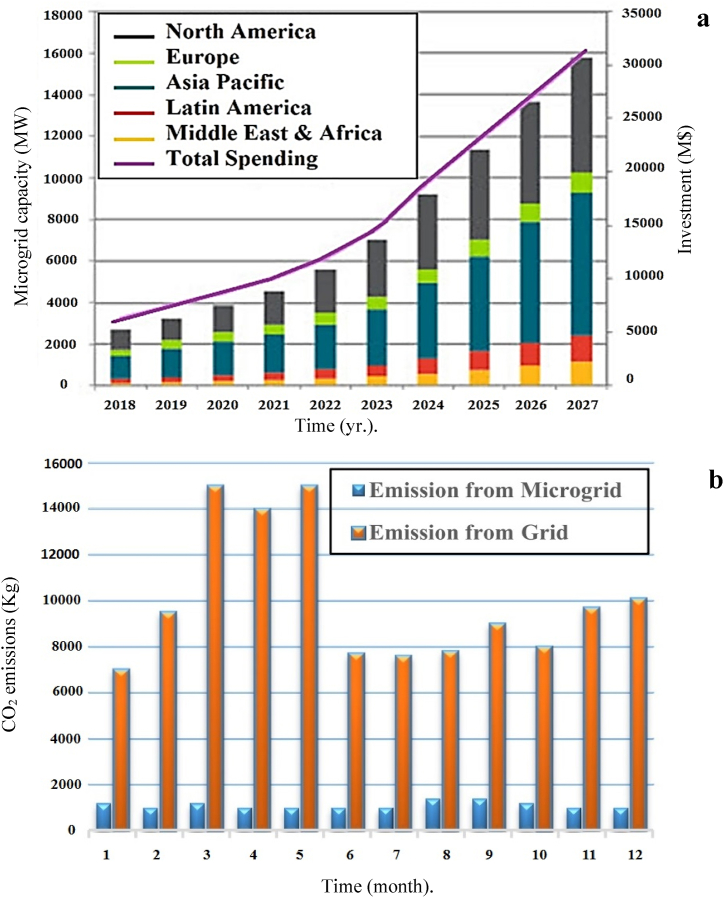
a) Global microgrid capacity and investment, b) Global CO 2 emissions from conventional power plants vs. microgrids in 2021 [ 12 ].
Microgrids have considerable advantages over conventional power generation units. As illustrated in Fig. 2 b, microgrids emit much less CO 2 than conventional power plants because they can deploy zero-emission energy sources for power generation. Also, microgrids can integrate clean distributed generation sources such as solar and wind energy with other sources such as natural gas, provide high-reliability power, use waste heat, and reduce greenhouse gas emissions and CO 2 . Microgrids can generate power continuously and sustainably for areas that do not have access to the grid, and the electricity produced by microgrids is often environmentally friendly. Microgrids can also meet the required power during a power outage [ 8 ]. Distributed generation and microgrids have lower installation and maintenance costs than traditional power plants. In addition to the disadvantages mentioned above regarding the use of conventional energy and fossil fuels for electricity generation, non-renewable resources, from the point of view of energy security and supply chain, also face many problems. In the last few months, the war between countries and the Middle East's insecurities clearly showed that fossil fuel energy security is not sustainable [ 9 , 10 ]. Factors such as changes in government policies, war, and sanctions have caused the security and supply chain of fossil fuels to be seriously affected, and the resilience and stability of the energy supply in the world have declined. Because of the problems with traditional power plants, their higher installation, development, and maintenance costs, and the benefits of using renewable resources and microgrids, clean microgrid technology has been used more and more in recent years and has caught the attention of investors [ 11 ].
Until recent years, distributed generation (i.e., wind turbines and photovoltaic panels) and renewable sources (i.e., bioenergy) were used only as backup power generation sources and were not considered the primary energy source [ 13 ]. In addition, due to the unpredictable nature of renewable energy sources, these sources have low reliability, leading to disrupting the balance and stability of the power generator unit. These issues caused comprehensive and detailed studies on the role of renewable resources in microgrids, especially biofuels produced from biomass, to be ignored and rarely found in the literature. However, global energy policies are moving towards decarbonization and using renewable energy sources and clean fuels ( Fig. 3 ). Integrating microgrids with distributed generation and green fuels (i.e., biofuels and bioenergy carriers) can play a significant role in achieving decarbonization goals [ 14 ]. In green microgrids, support units are usually used to generate power when renewable energy sources cannot provide the necessary power due to their intermittent nature. In fact, microgrid support units have the task of compensating for the lack of required electricity when distributed generation units cannot produce electricity, or in periods of overload and peak load, they enter the required power into the power grid [ 11 ]. Most of the supporting units of microgrids are diesel generators. Diesel generators generate power by burning fossil fuels such as diesel. Clean fuels should substitute for fossil fuels to reduce the consequences of climate change [ 15 ].
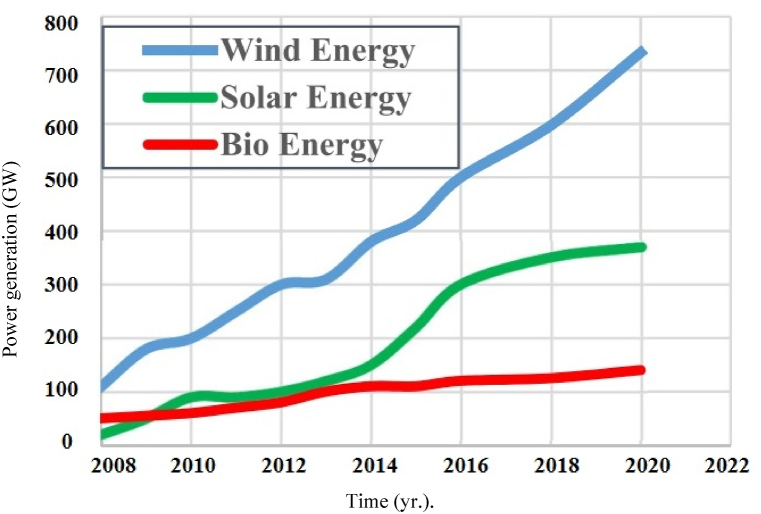
Global trend power generation from renewable sources [ 2 ].
Using renewable resources has prompted the global community to use green microgrids and renewable energy sources. Biofuel is one of the renewable energy sources that has attracted the attention of investors and researchers. The United States Department of Energy (DOE) has stated that biofuels burn cleaner than fossil fuels, produce fewer greenhouse gas emissions, and are completely biodegradable, in contrast to some fuel additives. It is estimated that cellulosic ethanol could decrease GHG emissions by at least 86% [ 16 ]. The availability of biomass and suitable infrastructure for the generation of biofuels and advanced technologies has led to the rapid development and extensive use of green fuels in microgrids in recent years [ 17 ]. Biofuels can be a suitable alternative to fossil fuels as low-carbon fuels because they mitigate greenhouse gas emissions and the impact of climate change caused by power generation. For this purpose, researchers have focused on increasing production and improving the efficiency of the production process for biofuels. Atikah et al. presented a method to increase the efficiency of biogas production. According to the study's findings, lipid-extracted Nannochloropsis gaditana (LEA) is appropriate for feedstock in gasification for synthesis gas production because LEA gasification produces a significant amount of H 2 while requiring little activation energy [ 18 ]. Pittman et al. showed that algae cultivation alone could not be cost-effective for biofuel production. They suggested that dual-use microalgae cultivation for biofuel production along with wastewater treatment can be an effective solution in terms of reducing greenhouse gas emissions and the cost of biofuel production. The high biomass productivity of microalgae cultured in wastewater indicates that this approach is a suitable and affordable method for biofuel production [ 19 ]. Also, several power plants worldwide currently use biofuels to produce power. Isabela, an island in the Galapagos, has operated since 2018 with a hybrid power supply system consisting of a solar plant, biofuel generators, and batteries. In addition, the Oak Ridge North, Texas, power plant operates three diesel generators entirely on biodiesel, a fuel derived from vegetable oil or animal fat, both of which have little sale value. However, biofuel production is associated with challenges and limitations, which cause the share of renewable energies to be about 13% of the total global energy consumption, which is about 10% for bioenergy [ 20 ]. In a study on biofuel production from algae, Hannon et al. concluded that this biofuel has the advantages of clean fuels that were mentioned, but the production of this type of fuel is associated with challenges such as the lack of suitable infrastructure, it is more expensive than conventional fuel, and the fact that it uses older technology [ 21 ]. Therefore, this review article attempts to study biofuels' role in improving microgrids' sustainability and reducing greenhouse gas emissions and climate change. The definition and classification of microgrids and their technical aspects are detailed. The characteristics of biofuels and their categories are also reviewed. In addition, the microgrids connected to the support unit with biofuels are discussed. Finally, limitations and future directions are examined. Table 1 lists some important research on the utilization of biofuels in microgrids compared to the present study.
Most important review papers regarding the use of biofuels in power generation systems.
2. Microgrids
To evaluate the role of biofuels in the sustainability of microgrids, it is necessary first to get acquainted with microgrids in detail. Therefore, in this section, the concept of microgrids will be explained first, and then the characteristics of microgrids will be discussed in detail. The following will examine the classification of microgrids and their technologies.
Many compliments can be found in the literature for the microgrid as a power generation unit. The schematic of a microgrid is shown in Fig. 4 a. The US Department of Energy provides a definition for microgrids that is widely cited by energy scientists and researchers. Experts and researchers of the US Department of Energy have identified the microgrid as a group of interconnected loads, and within the electrical boundaries, energy sources are distributed [ 26 ]. This group is considered a unit that can be controlled and operated according to grid conditions. Microgrids are connected to or disconnected from the grid. They always operate based on three fundamental principles, which are 1- Every part of the distribution system of a microgrid can be identified differently from the rest of system. 2- All sources connected to a microgrid should be controlled in their interaction and coordination. 3- Regardless of whether a microgrid is connected to a grid or not, it must be able to perform work and generate power [ 7 ]. This comprehensive definition, which researchers in the field of energy have widely used, does not mention the types of technologies that can or should be used in microgrids, nor does it mention anything about the size of distributed energy resources, their technical specifications, and sustainability [ 27 ]. Combined heat and power (CHP) systems, also known as cogeneration systems, are widely used in microgrids. Cogeneration systems meet the demands of heat and electricity loads. Fig. 4 b illustrates the general structure of a common CHP [ 28 , 29 ].
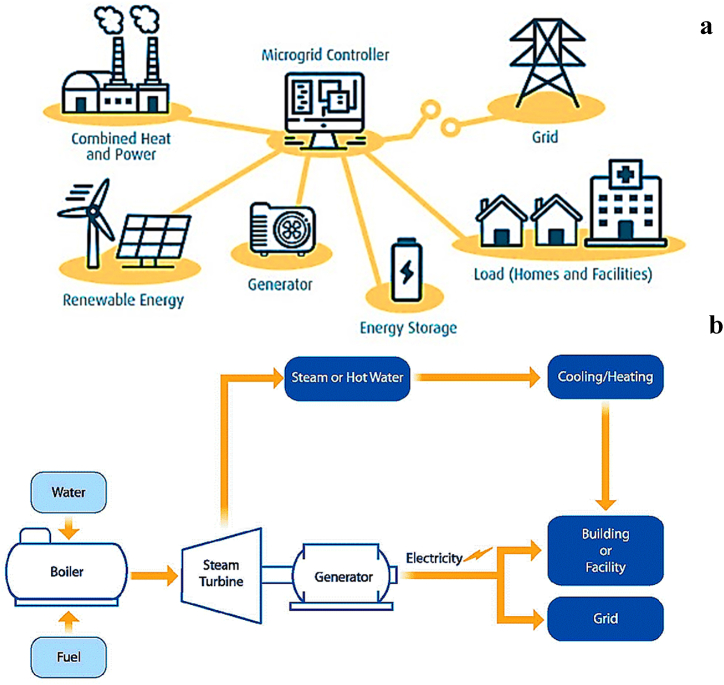
a) Schematic of a common microgrid [ 39 ], b) General structure of a common CHP [ 28 ].
Microgrids have different characteristics. One of the most prominent features of microgrids is power generation and storage. Many interdisciplinary studies include a wide range of power generation and storage sources in microgrids [ 30 , 31 ]. Many researchers consider microgrids as an agnostic technology, which means that in the design of microgrids, economic considerations and technical requirements of the project play a preeminent role. Most of the microgrid generation sources lack inertia, which is required by generators. Hence, a buffer is always used to reduce the adverse effects of imbalance in demand and power generation in microgrids [ 32 , 33 ]. At some times, microgrids are faced with a lack of production load, in which case energy storage technologies play a substantial role in the balance and resilience of microgrids [ 10 , 34 ]. Apart from electricity storage in microgrids, mechanical and thermal energy storage are also considered if needed. In microgrids, besides analyzing and checking the ability of storage units to support the power generation system and proper and optimal power storage, side tasks of storage units (i.e., voltage control support, peak load shaving, and load tracking) are also considered, and investigated [ [35] , [36] , [37] , [38] ]. However, evaluating the efficiency of the energy storage units used in microgrids has been neglected from an environmental and sustainability perspective.
In order to evaluate the performance of microgrids more precisely, it is necessary to classify these power generation units. Hence, researchers use various methods to classify microgrids. The most important and widely used classification of microgrids, which researchers often cite, is the microgrid classification based on the type of connection to the national power grid. From this point of view, scientists divide microgrids into two categories: microgrids connected to the grid and microgrids disconnected from the grid [ 40 , 41 ].
Grid-connected microgrids are connected to the national power grid [ 42 , 43 ]. When the local power generation units and microgrids cannot provide power, the required electricity is supplied through the national power grid, and the lack of produced electricity is provided through the grid. When the power generation units produce excess power, the control unit directs the excess electricity to the national electricity grid. In better words, microgrids interchange electrical energy with the grid when connected to the grid [ 44 , 45 ]. Fig. 5 a depicts a microgrid connected to the grid. The development of this type of microgrid will be costly, especially for remote areas. Also, grid-connected microgrids have high maintenance costs. More than a third of the world's population resides in remote and rural areas [ 46 ]. Using grid-connected microgrids to supply their electricity needs is either impossible or very difficult and expensive.
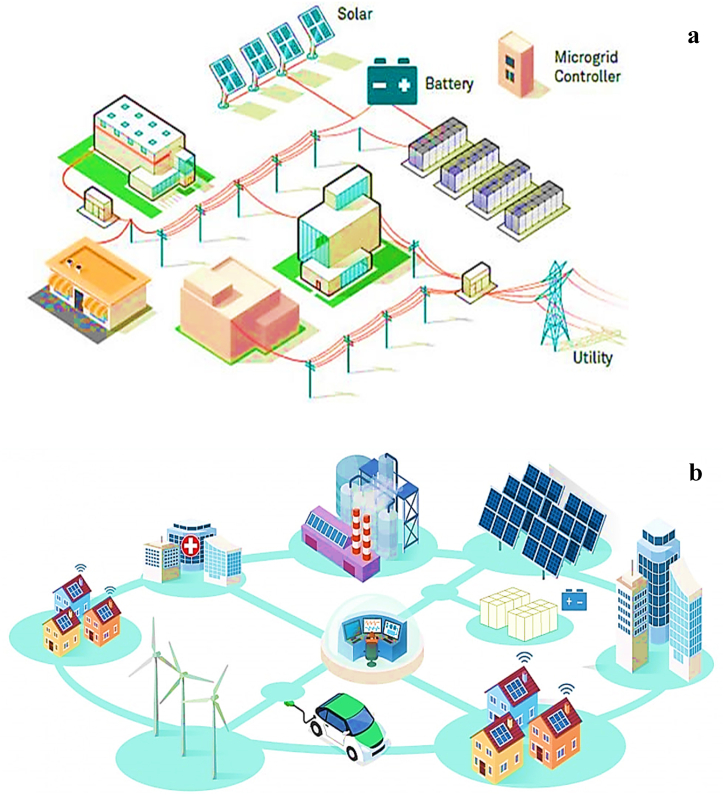
a) Schematic of a common on-grid microgrid, b) Schematic of a common islanded microgrid [ 11 ].
Stand-alone microgrids or islanded microgrids are other types of microgrids. In islanded microgrids, power generation is done from local sources. Stand-alone microgrids are not connected to the national power grid. Suppose the power generation units of the microgrid generate excess electricity. In that case, the control unit directs this excess electricity to the energy storage units and stores the extra power in the energy storage units. When the power generation units are facing a peak load or lack resources, it is possible to use the electricity stored in the storage units. The next characteristic of stand-alone microgrids is using a “support unit,” which is usually a diesel generator, besides using the energy storage unit [ 9 , 47 ]. Islanded microgrids can produce power in a place close to the power consumption, which decreases energy transmission losses. There is no need to develop and expand power grids in remote areas, thus significantly reducing the cost of power generation and transmission. The schematic of an islanded microgrid is shown in Fig. 5 b.
Significant factors such as environmental effects should also be considered in the classification of microgrids. For example, microgrids can be classified into two categories from an environmental point of view: green microgrids and conventional microgrids. Green microgrids generate power from renewable energy sources and cause the least environmental and human health damage. Biofuels derived from biomass could also be used in green microgrids as support units. In contrast to green microgrids, conventional microgrids use traditional sources and fossil fuels to produce electricity and cause the production of air-polluting gases. Table 2 lists some literature about microgrids considering environmental and human health aspects.
Some studies regarding power generation systems considering environmental and human health aspects.
3. Biofuels
As mentioned in the previous sections, one of the most crucial components of microgrids is the support units and energy storage systems. Nowadays, diesel generators are often used to compensate for the power deficit of distributed generation sources. Diesel generators have caused issues such as pollutant gas emissions, climate change, and depletion of fossil fuel resource reserves [ 62 ]. Therefore, scientists have conducted extensive research to find a suitable alternative to fossil fuels in generators used in microgrids. Bioenergy and biofuel have been introduced as the best options to deal with the above issues. Biofuel technologies use methods analogous to those employed with fossil fuels to generate heat and electricity from biomass. To release heat and energy from biomass, it can be burned, degraded by bacteria, or converted to a gas or liquid fuel. Biofuels are refined plant biomass products used for combustion and thermal energy production. Biofuels are also classified in terms of feedstock types for production. First, second, third, and fourth-generation biofuels are the different forms of biofuels. They are identified by their biomass sources, limitations, and technological advancements. The primary disadvantage of first-generation biofuels is that the feedstock from which they are made is a food source (wheat, corn, barley, etc.). Second-generation biofuels are made from non-food biomass (agricultural lignocellulosic waste, municipal solid waste, etc.), but they still compete for available land to produce the first-generation's feedstock. Third-generation biofuels are a better option for alternative fuel than first- and second-generation because they do not compete with food. Nevertheless, producing third-generation faces commercial difficulty. The combination of genetically modified feedstock and microorganisms that have been genomically prepared results in fourth-generation biofuels. Even though this biofuel is a well-known alternative to fossil fuels, the possible environmental and health risks of producing it are profound [ 63 ]. The history of using bioenergy and biofuels is as old as the history of humankind. Biofuels were used for household purposes like using wood and coal for heating houses, lighting, and cooking food [ 64 , 65 ]. Until the 19th century, biofuels such as wood and vegetable oils were used as the world's most significant source of fuel for heating and lighting. However, these fuels have given their place to exhaustible fuels. Fossil fuels dedicate the most extensive share of the world's energy supply. Nevertheless, the disadvantages of using exhaustible fuels have propelled governments, experts, and policymakers to use biofuels and bioenergy [ 20 ]. As shown in Fig. 6 a and b, global demand and production of biofuel will increase, highlighting the significance of biofuel's share in the future of the world energy market. Regardless, of the growth in the demand for and production of biofuels, the production of clean fuels is facing restrictions that will be discussed in the following. Also, in the next section, primarily common biofuels will be evaluated in detail.
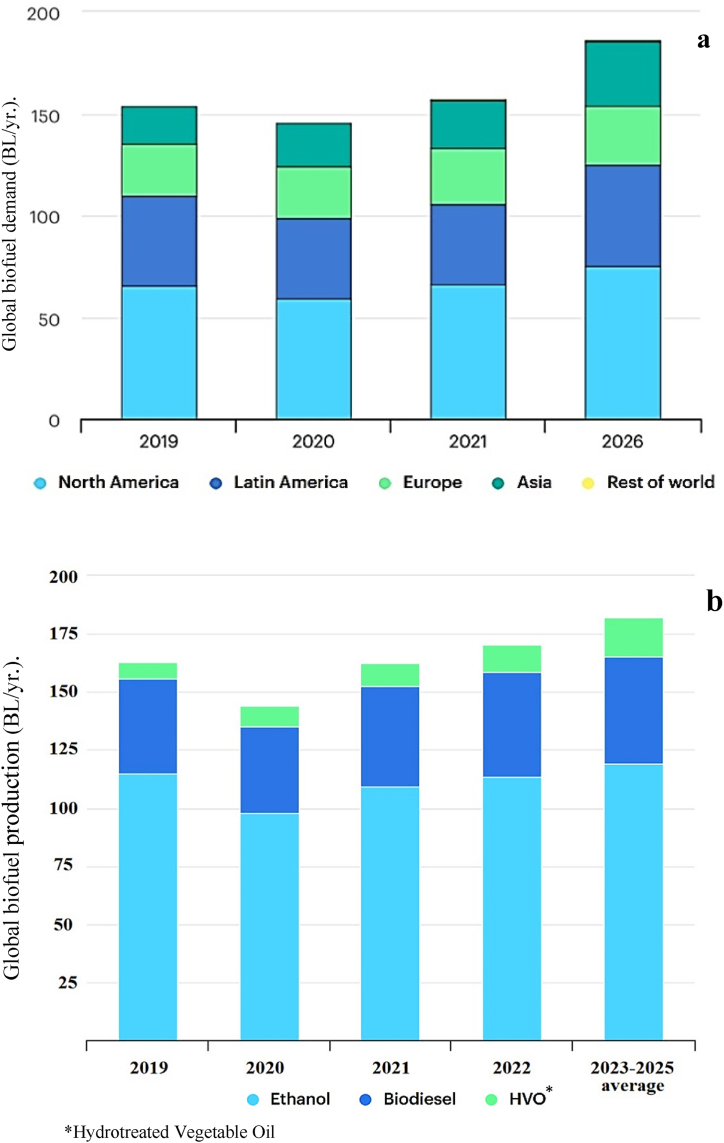
a) Global biofuel demand by region, 2019–2026, b) Global biofuel production in 2019 and forecast to 2025 [ 66 ].
3.1. Classification of biofuels
Today's advanced technologies can extract biofuels in different forms. Regarding availability form, biofuels are classified into three categories: solid, liquid, and gaseous [ 24 ]. Each of these categories will be discussed in detail in the following.
3.1.1. Solid biofuels
Solid biofuels are fuels produced from organic materials, biomass, and urban waste. Solid biofuels can be used to produce thermal and electrical energy. These renewable fuels are obtained from various sources, such as agricultural and forest residues and animal remains. The most famous solid biofuels are firewood, wood chips, wood pellets, and charcoal [ 67 ]. In the following section, the application, different forms, advantages, and disadvantages of using some widely used solid biofuels are discussed in detail.
3.1.1.1. Firewood
Wood and plant materials can be considered one of the oldest biofuels after human emergence. Before the advent of fossil fuels, people used firewood for heating and household purposes. Most firewood and plant materials' ignition temperatures are between 200 and 300 °C [ 68 ]. Thermal energy and light are released from burning firewood. By studying the literature, it will be apparent that since six thousand years ago, humankind has been able to use fire in a controlled manner for its purposes and needs. However, some research by archaeologists shows that the use of energy released from firewood is related to a million years ago. The thermal energy of firewood has also been used for melting ore, lighting roads, and making bricks and tiles. Firewood was traded in bulk, and the moisture content of firewood has a pronounced effect on the amount of energy emitted and its ignition temperature. Dry firewood usually has a moisture content of 10–25%. However, green or wet firewood usually has a moisture content of 40–100%, and in terms of energy content, it is less than dry firewood [ 69 ]. The primary reason for the low energy content of fresh firewood is that more heat must be consumed during combustion to convert the moisture content of green firewood into steam. For this reason, fresh firewood or green firewood is kept for six months in a place equipped with ventilation systems so that its humidity is less than 20% [ 70 ]. Firewood is currently used as the dominant fuel in many remote and rural areas in developing countries in Asia and Africa. According to researchers' estimates, about 2.6 billion people (40%) of the world's population rely on firewood to meet their needs, i.e., the people of the world consume about 1730 million cubic meters of firewood annually [ 71 ]. Even though, in recent decades, research reports a 3% growth in firewood consumption, the share of firewood in the total energy consumption in the world has been declining. As forecasted, firewood consumption as biofuel will be almost constant in the coming years. However, firewood cannot be a good alternative to fossil fuels in microgrids. The energy content of dry firewood is less than fossil fuels, and it is approximately 30–50% of the energy content of fossil fuels. For example, the energy content of a type of dry firewood called “well-seasoned firewood” is about 15 MJ/kg. After the complete combustion of firewood, thermal energy and light are released. This combustion also produces water vapor and CO 2 . One of the prominent drawbacks of using firewood is that it produces smoke, which is a mixture of volatile organic compounds, black carbon particles, and smoke condensate, harming the environment and human health. Burning firewood will also emit pollutants and toxic gases (i.e., methane, sulfur oxide, nitrogen oxides, and carbon monoxide) [ 72 ]. The pollutant gas emissions are mostly related to the incomplete combustion of firewood due to the lack of sufficient oxygen and low temperature. Researchers have estimated the combustion efficiency of firewood to be 80–85% when burning in a combustion chamber like a furnace.
3.1.1.2. Wood chips
Direct use of the heat and light released from burning firewood is the simplest and most efficient way to use thermal energy from biofuels because it does not require any other processing or operation. Since firewood is stored in bulky scales, using firewood involves a lot of space, which is unsuitable for homes and small spaces. Wood chips solve this problem [ 73 ]. Wood chips are small pieces of wood that result from cutting branches and tree trunks with wood chippers. Wood chips are also used for ornamental purposes, sports fields, and wood pulp production. In today's era, the use of wood chips to generate electricity and heat has grown significantly [ 68 ]. In developing countries, using firewood is still more common in rural and remote areas because the equipment and tools required for chopping wood are not available in these areas. While in advanced countries like the USA, wood chips are available to villagers at a cheaper price than firewood. Researchers propose using wood chips to produce thermal and electrical energy to mitigate the carbon footprint. For example, about three-quarters of the annual heat and hot water needed by Colgate University in America are provided by wood chips. For this purpose, 20 thousand tons of wood chips are burned annually. Wood chips decrease the heating costs of the Cayuga Nature Center in New York by 15,000 dollars annually. Also, the United States has approximately 222 power plants whose fuel is wood chips [ 74 ]. These power plants produce 7.5 billion kWh of electricity annually [ 75 ]. According to the US Department of Energy reports, in 2020, the United States obtained 1.42% of its electricity requirements from wood chips, equivalent to 57.6 billion kilowatts. Global bioenergy production capacity in 2020 was about 145 GW [ 76 ]. Wood chips emit fewer sulfur oxides and NOx than fossil fuels like coal when burned. Considering the problems of rising environmental pollution, climate change, policy and global requirements for the environment, as well as the tendency to use bioenergy, it is predicted that the use of wood chips will grow increasingly in the future [ 77 ]. However, wood chips lose significant energy value and dry matter weight during storage. This issue makes wood chips' efficiency lower than fossil fuels like diesel. In this regard, during the storage process of wood chips, material torrefaction is usually used to minimize the deterioration of wood chips. Torrefaction means heating wood in the absence of air at a temperature of 240–300 °C for partial pyrolysis. Nevertheless, this process will decrease up to 20% of the dry mass of the wood chips and 10% of their energy value but will guarantee a sterile, dry, stabilized, and hydrophobic product.
3.1.1.3. Wood pellets
Wood pellets as a biofuel require more processing than wood chips. The wood chunks are first turned into sawdust by a hammer mill to produce wood pellets. Then, under the pressure of the perforated plates, the sawdust is turned into 2–3 cm long pellets. From the mechanical point of view, the pellets are durable, i.e., with each movement, only about 3% of the pellets become smaller particles and are usually light brown. The unique characteristics of the pellets, such as the appropriate shape, small size, low moisture content, and high density, make the pellets very suitable for long-distance transportation and storage in warehouses. Since wood pellets are more expensive than wood chips, their use for household purposes is much more limited than wood chips [ 78 , 79 ]. The global production of wood pellets in 2020 was more than 45 million tons. This amount is expected to increase by 2025 [ 80 ]. However, the growth in the production of pellets in the world, especially in countries like the USA, has worried environmental experts because producing pellets has destroyed many wetlands and forests. Moreover, the production cost of the pellets is high. For affordable production of pellets and a move toward a green economy, the technology of producing pellets should be improved.
3.1.1.4. Charcoal
Wood biofuels such as wood chips, firewood, and wood pellet have a lower energy content than fossil fuels such as diesel, but their combustion emissions are higher than fossil fuels. The low firing temperature of wood fuels (less than 850 °C) cannot be used for industrial purposes such as metal melting. Therefore, engineers and scientists used the technique of transforming wood into charcoal [ 81 , 82 ]. The pyrolysis technique produces a solid, carbon-enriched, and porous material. Wood materials are heated in chambers up to 400 °C in an oxygen-depleted environment to transform them into charcoal. The energy content of charcoal is higher than coal and is usually 28–33 MJ/kg [ 83 ]. Charcoal burns without smoke and flame. Charcoal is capable of producing heat up to 2700 °C. Charcoal is usually produced as briquettes for commercial purposes and for more convenient transportation. During the Second World War, when the world faced a gasoline shortage, many cars were supplied with wood gas and a mixture of hydrogen and carbon monoxide generated by burning charcoal in a chamber [ 84 ]. Charcoal is also used for domestic cooking and purifying air and water. The global production of charcoal per year is about 51 million tons, which is expected to remain at this level in the coming years [ 85 ]. The high cost and the complex process of charcoal production are two limiting factors for the development of using charcoal in industries such as the power generation industry.
3.1.2. Liquid biofuels
Any renewable fuel in liquid form is referred to as liquid biofuel. Liquid biofuels are primarily used in the transportation industry and power generation. In recent years, biofuel production has proliferated ( Fig. 7 ). The amount of biofuel produced reached 96 Mtoe in 2020, from 8.57 Mtoe in 1990 [ 86 , 87 ]. Bioethanol, biodiesel, and pyrolysis bio-oil are notable examples of liquid biofuels. Liquid biofuels are popular because of their unique features, including safer transportation, relative affordability, storage stability, high energy-to-mass ratio, high combustibility, and lower greenhouse gas emissions than fossil fuels [ 88 , 89 ]. The most widely used liquid biofuels are bioethanol, biodiesel, and pyrolysis bio-oil [ 67 ]. In this section, the application, benefits, and drawbacks of using these kinds of liquid biofuels are examined in detail.
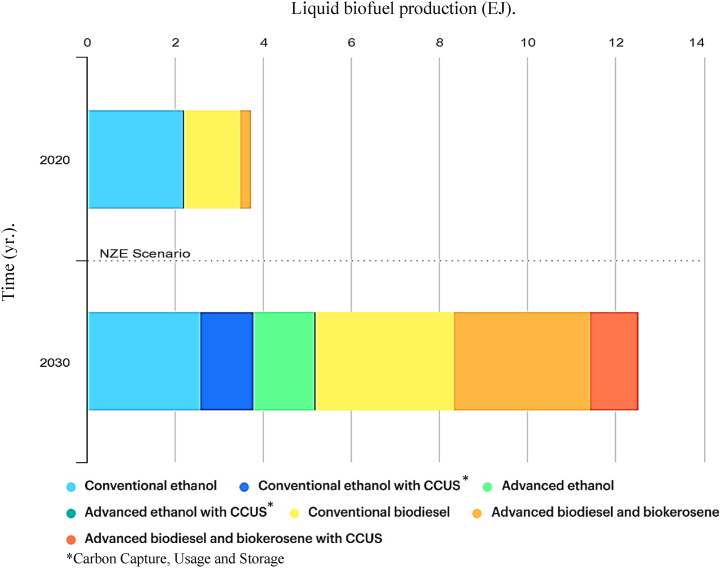
Liquid biofuel production in 2020, and in the Net Zero Scenario in 2030 [ 66 ].
3.1.2.1. Bioethanol
Ethanol can be derived from the fermentation of simple sugars such as glucose and fructose from plant biomass. As shown in Fig. 8 , the main ingredients of plant cell walls are hemicellulose, cellulose, and lignin. However, depolymerizing cellulose and hemicellulose and converting them into simple sugars is not an easy task. Researchers tend to develop pretreatment technologies and find practical solutions to obtain simple sugars from lignocellulosic materials [ 90 ].
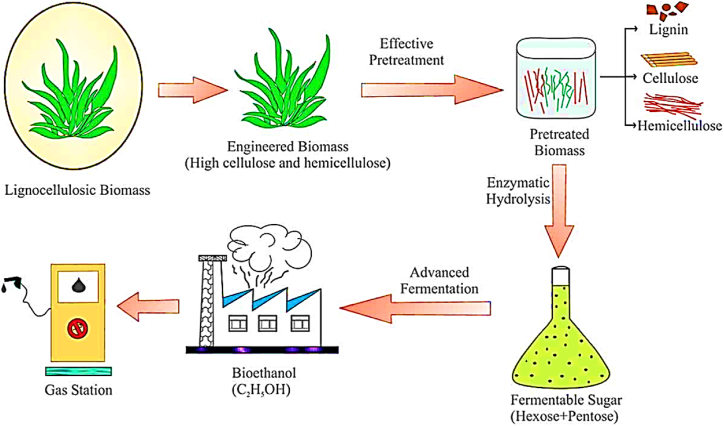
Bioethanol production process (Lamichhane et al., 2021).
Today, most commercial bioethanol is produced from crops such as sugarcane, beet, wheat, corn, and potatoes. The largest producers of bioethanol in the world belong to the countries of America, Brazil, Europe, China, and Canada, respectively. The United States allocates an average of 114 million tons of harvested corn kernels annually, about 42% of the whole corn kernels, to bioethanol production [ 91 ]. These kinds of biofuels are renewable and clean fuels, but production of these types of biofuels faces various challenges, such as increasing food prices, reducing global food security, competition for land and water, and other issues related to the food vs. fuel dilemma. Due to the drawbacks of these kinds of biofuels, they cannot be considered an alternative to fossil fuels.
Researchers conducted extensive research on using non-food lignocellulosic plant materials to reduce the adverse effects of bioethanol production from food products [ 92 ]. However, this category's crops require fertilizer and pesticides, and their production is not invariably eco-friendly. This biofuel also requires complex and expensive technologies for production and is not economical from an economic point of view, so it has faced a significant commercialization obstacle. Some bioethanol companies in America, Canada, and Spain using new and advanced technologies in the pretreatment of acid/enzymatic hydrolysis and biomass increased bioethanol productivity to 83 gallons per ton of biomass [ 93 ] and caused an increase in the consumption of biofuels in the world. For example, bioethanol consumption in the United States of America has increased from 4.7 billion gallons in 2007 to 36 billion gallons in 2022 [ 94 ].
3.1.2.2. Biodiesel
Diesel is a fossil fuel obtained from the distillation of crude oil at a temperature of 200–350 °C in refineries. The energy content of diesel is higher than gasoline [ 95 ]. This liquid hydrocarbon fuel is the dominant fuel of power plants worldwide. Due to the decline of world oil reserves, liquid biofuels such as biodiesel are a clean and suitable alternative to diesel. It is obtained by transesterifying animal fats, vegetable oils, waste fats, and algal lipids in the presence of alkaline catalysts and alcohol [ 96 ]. The energy density of diesel is almost 90% of petrol diesel, equal to 38–45 MJ per kilogram. George Chavanne [ 97 ], one of the Belgian researchers and scientists, registered the method of using vegetable oil to produce biodiesel in 1937. His innovative approach used the transesterification (alcoholics) process to break down fat molecules to produce biodiesel. Years later, a scientist from Brazil developed and expanded the industrial process of biodiesel production. The sudden increase in oil prices in 2001 and the reduction in energy security in those years made biodiesel a popular fuel in the world energy market, so biodiesel production reached more than 6 million gallons in 2013 [ 96 ]. Animal fats, algal lipids, vegetable oils, and oilseed plants are excellent sources for biodiesel production. The standard methods of biodiesel production are shown in Fig. 9 . In the past few years, significant progress has been made in identifying algal or plant species with high oil yields, optimizing biodiesel production methods, and specifying the characteristics of crude oil on which biodiesel quality depends [ 98 ]. Global biodiesel production and consumption are steadily increasing, rising from 213 million gallons in 2000 to over 8500 million gallons in 2022 [ 99 , 100 ]. The largest biodiesel-producing countries in the world are the European Union, Brazil, Argentina, the United States, and China, respectively. Biodiesel has benefits like ease of use, simplicity of production, and cleaner emissions. The experts predict that the growing trend of biodiesel production will be maintained [ 101 ].
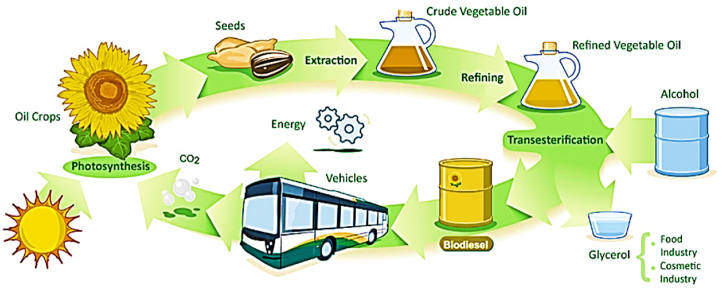
The biodiesel life cycle [ 102 ].
As mentioned, using biodiesel instead of fossil fuels in various industries, such as power and heat production, has advantages. However, biodiesel production faces obstacles and limitations that must be overcome. For example, it requires a lot of energy to produce biodiesel from crops, as power is needed for fertilizing, sowing, and harvesting crops. Besides this, the raw material must be transported, which consumes additional fuel. In other words, producing one gallon of biodiesel requires an energy counterpart to several gallons of fossil fuel. In addition, biodiesel contains approximately 10% more nitrogen oxide (NOx) than conventional petroleum products, which causes acid rain. Moreover, biodiesel cannot be utilized at low temperatures and will gel at freezing temperatures. Also, biodiesel is more expensive than other conventional fuels.
3.1.2.3. Pyrolysis bio-oil
Exhaustible fuels were formed millions of years ago from the remains of plants and animals under the pressure and heat of the earth. Researchers made many efforts to obtain fuels similar to fossil fuels from plant biomass under similar conditions and at high pressure and temperature in an oxygen-free environment. However, their efforts were not fruitful [ 103 , 104 ].
The process of heating plant biomass at a temperature of 300–900 °C and in an environment free of oxygen is called pyrolysis. About five thousand years ago, the Amazon natives used this technique to produce biochar, and the ancient Chinese also used this method to convert wood into charcoal [ 105 , 106 ]. The pyrolysis technique of plant materials provides three products: bio-oil, synthesis gas, and biochar ( Fig. 10 ). According to the heat rate used in the pyrolysis technique, there are two methods for performing pyrolysis: fast and slow [ 107 ]. Today, the technique to transform pyrolysis bio-oil into a commercial and affordable fuel for industrial usage is still under investigation. Other uses of pyrolysis bio-oil include applying preservatives, lubricants, thickeners, adhesives, stabilizers, binders, etc. Although experts have an optimistic perspective on pyrolysis bio-oils, this fuel cannot be called an alternative to fossil fuels [ 108 , 109 ].
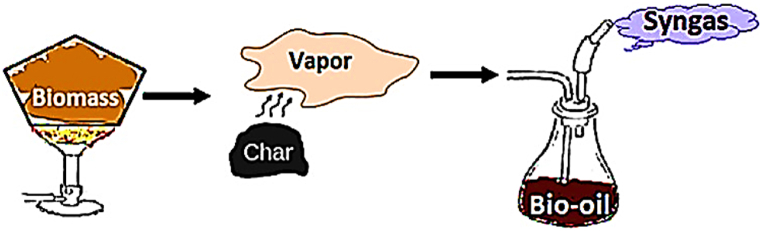
Plant biomass is pyrolyzed to produce syngas, bio-oil, and char [ 73 ]. With permission from Elsevier. Copyright©2014, License Number: 5378030157912.
3.1.3. Gaseous biofuels
Another category of biofuels is gaseous biofuels. It can be mentioned that biogas and syngas are among the most common gaseous biofuels. Gaseous biofuels have many applications, especially in the power generation industry and microgrids. Due to the many benefits of biofuels, in recent years, researchers have conducted extensive research to evaluate these fuels. The following section will review and introduce two common gaseous biofuels. Gaseous biofuels have advantages like less waste generation during use, ease of control, higher reactivity, and fewer oxidants demanded [ 110 , 111 ].
3.1.3.1. Biogas
Like other fossil fuels, natural gas is formed from the burial of animal and plant remains over thousands of years under high pressure and heat. A major part of natural gas is methane (CH 4 , 95%), and about 5% of natural gas consists of propane (C 3 H 8 ), carbon dioxide (CO 2 ), ethane (C 2 H 6 ), nitrogen (N 2 ), ethane, and butane (C 4 H 10 ) [ 112 ]. Natural gas was first used for street lighting in 1821 in New York. Natural gas is used in various industries, such as electricity generation, transportation, and cooking. The United States and China are the largest producers and also the largest consumers of natural gas in the world [ 113 ]. Gaseous fuel obtained from renewable materials is called biogas. Biogases are a suitable alternative to natural gases and are produced from the process of anaerobic digestion of organic materials. Biogas contains a small amount of water vapor, hydrogen, and H 2 S, along with methane (60–65%) and carbon dioxide (30–35%). The decomposition of biomass by microorganisms in the absence of oxygen is called anaerobic digestion [ 114 , 115 ].
About 120 cubic meters of biomethane can be extracted from one ton of biowaste, and this amount of biomethane is capable of producing 200 kW h of electricity (Khalil et al., 2019). In 1808, an English scientist obtained methane from cattle manure. In 1859, the first anaerobic digestion plant was established in India. Nayono et al. [ 116 ] studied anaerobic digestion for optimal methane production to find the best anaerobic bacteria and conditions for anaerobic operation. Nowadays, the use of biogas has proliferated throughout the world. There are more than 4.5 million anaerobic digesters in India for biogas production. The Chinese government has distributed more than 3.5 million anaerobic digesters in rural and remote areas. Among European countries, Germany is the pioneer in the production of biogas power plants and has produced more than 18.2 billion kilowatt hours of electricity from biogas power plants [ 117 , 118 ]. Although biogas has accounted for a significant amount of energy production globally, most energy experts believe that this biofuel has a much greater potential to allocate a more substantial share of energy production globally. According to predictions, two countries, China and Germany, have tremendous potential for biogas production in the future [ 25 , 119 ].
3.1.3.2. Syngas
Among other biofuels in gaseous form is syngas. Syngas is produced from the thermal decomposition or gasification of plant materials. Syngas consists of 0–5% methane, 15‒5% carbon dioxide, 25–30% hydrogen, and 30–60% carbon monoxide. Syngas also contains a small amount of H2S and water vapor [ 120 , 121 ]. Fig. 11 shows how syngas is produced.
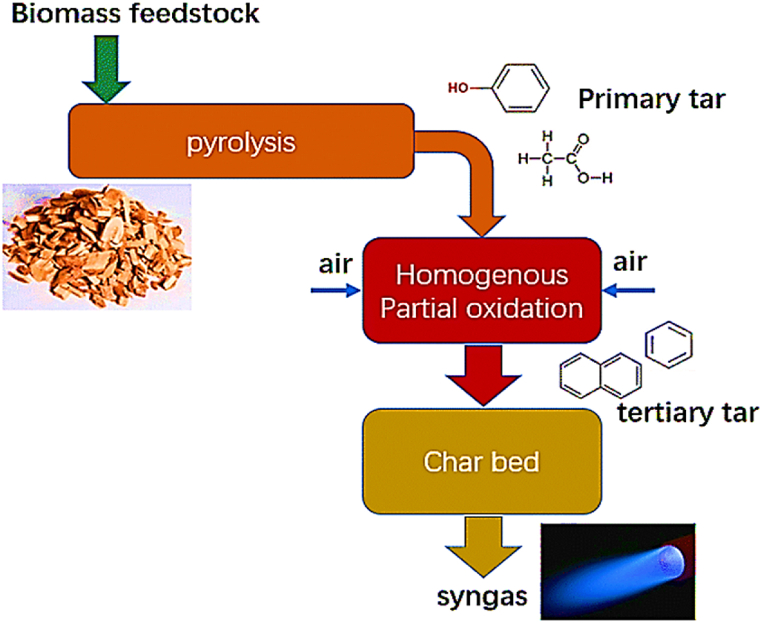
The process of syngas production [ 122 ].
The conversion rate of wood gasification to syngas is 92%. Also, the conversion rate of wood bioenergy to syngas energy is 62%, and the conversion rate of wood gasification to hydrogen is 71%. The density of syngas is approximately 5.3 MJ/Nm 3 , and 48% of syngas is nitrogen. It also contains H 2 S and CO 2 . Syngas is burned directly in power plants to produce electricity. Hydrogen separated from syngas is used to produce electricity from hydrogen fuel cells [ 123 , 124 ]. China, Europe, and the United States have the most significant number of commercial gasification plants worldwide. Approximately 11% of the capacity of the world's gasification plants is dedicated to electricity production [ 125 , 126 ]. Some strict requirements and policies for syngas production caused global gasification based on biomass to not develop as expected. More evaluations and studies need to be done to find economical, optimal, and efficient solutions for syngas generation. Table 3 summarizes biofuels' advantages, disadvantages, and applications in three categories: solid, liquid, and gas.
Comparing the advantages, disadvantages, and applications of solid, liquid, and gas biofuels.
4. Microgrids integrated with biofuels
As described in previous sections, the support unit is one of the vital elements in the microgrid system. The support unit plays a role when the distributed generation sources cannot supply the required load or when the microgrid is facing a peak load. Most of the fuel for the support units of traditional microgrids is supplied from fossil fuels, which were mentioned in detail with their drawbacks in the previous sections. This section deals with the latest research and projects on microgrids integrated with biofuel support units.
4.1. Microgrids integrated with solid biofuel
Solid biofuels, including firewood and charcoal, are one of the sources of biofuel in some microgrids or power generation systems. Sookramoon [ 127 ] proposed a power generation unit integrated with solid biofuel. The proposed solid biofuel was Pradauk biomass. The research results showed that the power generation system equipped with Pradauk biomass had a better performance than the power generation system equipped with coal. Also, this research indicated that no fuel supplement is needed for the smooth operation of power-generating engines. The power generation system equipped with solid biofuel was considered for rural areas of Thailand. Simanjuntak et al. [ 20 ] examined the progress and development of bioenergy as an alternative source of fossil fuels to meet the energy needs in Indonesia. Research showed that the Indonesian government's policies to support biofuels are improper; for example, the government's support for natural gas significantly reduced the consumption of solid biofuels for power generation. They suggested that government support for solid biofuels should be increased, and the government should support larger-scale industries to use more solid biofuels. Solid biofuels are well-developed and well-received for smaller-scale power generation. Simanjuntak et al. [ 20 ] also showed that if the government supports bioenergies and allocates a more significant contribution to bioenergies, especially solid biofuels, to meet energy demands, it will mitigate environmental concerns in Indonesia. Sapariya et al. [ 128 ] suggested generating electricity and heat using municipal solid waste and raw materials of Covid-19 waste through thermochemical gasification techniques. The energy-generated system from urban solid waste is an environmentally friendly approach and is a suitable alternative to fossil fuels. This research investigated the main effective parameters for increasing the efficiency of the heat and energy production system from municipal solid waste. Sikkema et al. [ 129 ] investigated how solid biomass can contribute to the European Union's renewable energy goals until 2030. The researchers' main goal was to determine the contribution of solid biofuels to the EU's energy supply in the coming years. The research results showed that the leading countries of the European Union, such as Germany and France, have acquired the goals of the 2030 European Union or are close to these goals, but the lagging countries are far from these goals. According to the research results, forest biomass or solid biofuel increases bioenergy sustainability in the European Union despite some challenges. Table 4 lists some studies that use solid biofuel for power generation.
Summary of some studies on solid biofuel for power generation.
As the study results have indicated, solid biofuels are an excellent source for producing bioenergy, power, and heat. Energy production from solid biofuels is cost-effective and environmentally friendly in countries with a high potential for solid biomass, like European countries, Southeast Asian countries, and Canada. Considering that solid biofuels can be a suitable alternative to fossil fuels, more studies should be made to increase the share of solid biofuels in the world's energy supply. The important point in using solid biofuels such as forest biomass is that their environmental stability and ecosystem should be maintained.
4.2. Microgrids integrated with liquid biofuel
Liquid biofuels are among the most widely used biofuels in power or heat production systems. As explained in detail in Section 3 , liquid biofuels have a good share of the power and heat supply system in many developed countries. Biodiesel, bioethanol, pyrolysis, etc., are widely used liquid biofuels. In a study by Latif et al. [ 138 ], a stand-alone microgrid consisting of a solar thermal system as a power generation unit and a generator as a support unit was evaluated. The fuel of the support unit was biodiesel. This research aimed to manage load frequency stabilization of the islanded microgrid to balance the load demand and supply. They used the firefly algorithm (FF) and particle swarm optimization (PSO) to optimize this island microgrid. Bhuyan et al. [ 139 ], proposed a new strategy to control a hybrid microgrid consisting of the wind turbine, energy storage unit, and solar panel. The proposed off-grid microgrid was equipped with a biodiesel generator as a support unit. The study results showed that the proposed strategy performed better than a traditional control system. Also, the use of biofuel had fewer environmental impacts than fossil fuel. Feroldi and Zumoffen [ 140 ], designed a hybrid microgrid system based on wind and solar renewable energy resources with an innovative method. The hybrid system mentioned included an energy storage unit and bioethanol. These researchers presented a new optimal sizing method considering energy management, which is economical and highly reliable. In a study led by Chiaramonti et al. [ 141 ], they investigated the feasibility and potential of using liquid pyrolysis as liquid biofuel instead of fossil fuels such as diesel and natural gas for power generation. Researchers focused on electricity generation from liquid pyrolysis derived from biomass, which was of great interest to experts and investors in natural gas/steam power plants or power plants equipped with gas turbines and diesel generators. The results indicated that due to the significant progress made in recent years in the field of biomass pyrolysis technology to produce electricity and the many advantages that liquid pyrolysis has over fossil fuels, there is still a long way to make use of this liquid biofuel economically. Table 5 summarizes the research conducted for electricity production using liquid biofuel.
Summarize the research conducted for electricity production using liquid biofuel.

4.3. Microgrids integrated with gas biofuel
Today, power plants that produce electricity and heat using gas biofuel have grown significantly. Gaseous biofuels such as syngas and biogas have taken part of the contribution of fossil fuels in power supply as clean biofuels in some countries with high energy consumption or industrial countries that produce large amounts of power. But the use of gaseous biofuels has not yet met the expectations and predictions of experts in the energy field. In research by Jabari et al. [ 67 ], they proposed a simultaneous water-power production system that uses a gas turbine to provide the necessary power for a water desalination unit. The gas turbine's primary fuel is biogas, a fuel biofuel. The proposed water and power production system has solved the water lack and reduced the emission of polluting gases. Yıldız et al. [ 155 ], optimized an off-grid microgrid using the Improved Salp Swarm Algorithm to meet the required load. The constituent components of the off-grid microgrid included the photovoltaic panel, biogas, fuel cell, electrolyzer, and hydrogen storage unit. When the power generation units produced excess power, the extra energy was directed to electrolysis to produce hydrogen and store it. The reformer converted the excess biogas fuel into hydrogen and stored it in hydrogen tanks. The proposed power generation system provided the required power continuously and with high reliability. Nikhil et al. [ 119 ], propose an optimal renewable microgrid using Honey Badger Algorithm. The hybrid renewable energy microgrid consisted of the solar photovoltaic, wind turbine, battery storage system, and biogas engine generator. Since distributed renewable generation sources are unpredictable to balance the relationship between generation and power demand, suitable controllers and good performance optimization methods were proposed. The use of gaseous biofuel mitigated the emission of polluting gases produced by the renewable hybrid microgrid. Table 6 lists some studies on energy production systems equipped with gaseous biofuel.
List of some studies on energy production systems equipped with gaseous biofuel.
As reviewed in this section, there are many power generation projects in the world that are integrated with and supported by biofuel sources. The rate of usage of biofuel is proliferating. Biofuels could be a promising source to promote clean energy and resilience in microgrids; thus, they play a crucial role in the future development of the energy market. Biofuel helps reduce power generation industries' carbon footprint, recycle waste products, and have a superior energy balance. Every gallon of biofuel that substitutes for a gallon of fossil fuel helps mitigate CO2 emissions and climate change.
5. Limitations and challenges
Although the need for biofuels is very high, biofuels can be the most promising alternative to fossil fuels. Using biofuels instead of exhaustible fuels brings many benefits to human health and the environment, but there are many challenges and limitations to achieving this target. These challenges will be discussed in this section from different perspectives.
Environmental challenges are one of the challenges of using biofuels. One of the vital issues that can be mentioned is the environmental and social effects of biofuel production. The increase in demand for biofuels leads to soil fertility problems, the loss of forests and biodiversity, and somehow causes an increase in carbon dioxide in the environment and atmosphere [ 171 , 172 ]. The cultivation of agricultural lands for the production of biofuels’ feedstock causes a decrease in the fertility and quality of the soil and leads to the soil lacking nutrients and minerals, which also causes a reduction in the availability of water in the soil for plants. To produce more biomass is necessary to use more insecticides and fertilizers, which leads to groundwater pollution, soil degradation, and human health damage [ 173 , 174 ].
From the production and economic point of view, the production of biofuels also faces limitations and challenges. The production of biogas is restricted due to the high costs of production and the absence of subsidies. The production of biomethane (more than 97% CH 4 ) by the natural gas grid is restricted in its ability to fulfill the demand for microgrids and transportation fuel. When biomass is used to produce syngas, there is frequently an absence of the CO, H 2 , and CH 4 components necessary to fulfill the gas heating value for the engine. The process of fuel production from biomass must be economical. The gap and price difference between conventional fuels and biofuels are still one of the biggest concerns for the development and sustainability of biofuel production. The flexibility of feedstock materials for the production of biofuels is another problem in biofuels production. The choosing and production of suitable types of feedstock materials for producing biofuels are still being discussed and investigated by researchers due to the tremendous progress [ 173 , 175 ]. The production process of biofuels is very dependent on feedstock materials. The production process must be efficient and compatible with the environment and human health. Since the production and selection of feedstock materials for biofuel production face challenges, the production process also faces limitations and challenges such as sustainability and compatibility [ 176 , 177 ].
In terms of distribution problems, biofuels also face obstacles. Storage of feedstock materials and biofuels, the infrastructure of biofuels, and the marketing of biofuels are the primary problems that must be overcome. The high costs of producing biofuels and the special conditions of their transportation and storage lead to issues in biofuel distribution [ 178 , 179 ].
The challenges facing the development and production of biofuels can also be examined from the perspective of sustainability and the availability of feedstock materials for biofuel production. The ideal feedstock for biofuel production has conditions such as affordability, easy availability, high yield, minimal need for fertilizer and water, and production with the lowest greenhouse gas emissions [ 180 , 181 ]. One of the most prominent concerns for biofuel production is the availability of feedstock materials in bulk at reasonable prices. When choosing the suitable type of biomass for biofuel production, feedstock materials that have a negligible negative impact on agricultural land and require minimal water consumption should be considered. Storage and thermal stability of biofuels are other challenges that researchers are still studying to overcome [ 182 ].
6. Conclusion and future directions
This study showed that liquid and gaseous biofuels such as biodiesel and biogas have a very high potential to replace fossil fuels in power and heat production systems. The desire to use liquid biofuels such as bioethanol is high, but the high costs of producing and maintaining this liquid biofuel have made them less preferred. The literature review showed that using waste materials, such as urban solid waste, plant remains, forests, algae, animal remains, etc., can be suitable and economical options as feedstock materials for producing biofuels. Utilizing local feedstock materials that are abundant and accessible locally, applying new technologies in the biofuel production process, enlisting the participation of international organizations, receiving the support and incentives of governments for biofuel production, and attracting investors will help ensure proper distribution and maintaining the sustainability of biofuel production. The results of this investigation indicated that advancement and improvement in bioenergy production technologies, especially biogas, could, in the future, solve the problem of providing clean and affordable fuels for power and heat generation plants. Also, the rising utilization of the new generations of biomass (fourth generation) to produce biofuel solves the issue of food, water, and soil conflicts. Furthermore, determining the potential of each region in the world to produce biofuel from the local biomass can be beneficial for future work. In addition, providing a policy or road map for each country to produce biofuel while considering environmental and economic aspects is an excellent way to move toward carbon neutrality.
Author contribution statement
All authors listed have significantly contributed to the development and the writing of this article.
Funding statement
This research did not receive any specific grant from funding agencies in the public, commercial, or not-for-profit sectors.
Data availability statement
Data will be made available on request.
Declaration of interest's statement
The authors declare no competing interests.
- 1. Suman G.K., Guerrero J.M., Roy O.P. Optimisation of solar/wind/bio-generator/diesel/battery based microgrids for rural areas: a PSO-GWO approach. Sustain. Cities Soc. 2021;67 doi: 10.1016/J.SCS.2021.102723. [ DOI ] [ Google Scholar ]
- 2. Renewable Capacity Statistics. 2017. /publications/2017/Mar/Renewable-Capacity-Statistics-2017 accessed. [ Google Scholar ]
- 3. Fan Z., Fan B., Peng J., Liu W. Operation loss minimization targeted distributed optimal control of DC microgrids. IEEE Syst. J. 2021;15:5186–5196. doi: 10.1109/JSYST.2020.3035059. [ DOI ] [ Google Scholar ]
- 4. Yoldaş Y., Önen A., Muyeen S.M., Vasilakos A.V., Alan İ. Enhancing smart grid with microgrids: challenges and opportunities. Renew. Sustain. Energy Rev. 2017;72:205–214. doi: 10.1016/J.RSER.2017.01.064. [ DOI ] [ Google Scholar ]
- 5. IRENA International renewable energy agency. 2022. https://irena.org/ accessed.
- 6. Palizban O., Kauhaniemi K. Microgrid control principles in island mode operation. IEEE Grenoble Conf. PowerTech, POWERTECH. 2013;2013 doi: 10.1109/PTC.2013.6652453. [ DOI ] [ Google Scholar ]
- 7. Martin-Martínez F., Sánchez-Miralles A., Rivier M. A literature review of Microgrids: a functional layer based classification. Renew. Sustain. Energy Rev. 2016;62:1133–1153. doi: 10.1016/J.RSER.2016.05.025. [ DOI ] [ Google Scholar ]
- 8. Bhagawath S., Rajan S.E., Bhagawath S., Rajan S.E. Managing of smart micro-grid connected scheme using group search optimization. Circ. Syst. 2016;7:3095–3111. doi: 10.4236/CS.2016.710263. [ DOI ] [ Google Scholar ]
- 9. Kiehbadroudinezhad M., Rajabipour A., Cada M., Khanali M. Modeling, design, and optimization of a cost-effective and reliable hybrid renewable energy system integrated with desalination using the division algorithm. Int. J. Energy Res. 2021;45 doi: 10.1002/er.5628. [ DOI ] [ Google Scholar ]
- 10. Kiehbadroudinezhad M., Merabet A., Abo-Khalil A.G., Salameh T., Ghenai C. Intelligent and optimized microgrids for future supply power from renewable energy resources: a review. Energies. 2022;15:3359. doi: 10.3390/EN15093359. [ DOI ] [ Google Scholar ]
- 11. Kiehbadroudinezhad M., Merabet A., Hosseinzadeh-Bandbafha H. Optimization of wind energy battery storage microgrid by division algorithm considering cumulative exergy demand for power-water cogeneration. Energies. 2021;14:3777. [ Google Scholar ]
- 12. Saeed M.H., Fangzong W., Kalwar B.A., Iqbal S. A review on microgrids' challenges perspectives. IEEE Access. 2021;9:166502–166517. doi: 10.1109/ACCESS.2021.3135083. [ DOI ] [ Google Scholar ]
- 13. Kiehbadroudinezhad M., Merabet A., Rajabipour A., Cada M., Kiehbadroudinezhad S., Khanali M., Hosseinzadeh-Bandbafha H. Optimization of wind/solar energy microgrid by division algorithm considering human health and environmental impacts for power-water cogeneration. Energy Convers. Manag. 2022;252 doi: 10.1016/j.enconman.2021.115064. [ DOI ] [ Google Scholar ]
- 14. He Y., Guo S., Zhou J., Ye J., Huang J., Zheng K., Du X. Multi-objective planning-operation co-optimization of renewable energy system with hybrid energy storages. Renew. Energy. 2022;184:776–790. doi: 10.1016/j.renene.2021.11.116. [ DOI ] [ Google Scholar ]
- 15. Abazari A., Soleymani M.M., Kamwa I., Babaei M., Ghafouri M., Muyeen S.M., Foley A.M. A reliable and cost-effective planning framework of rural area hybrid system considering intelligent weather forecasting. Energy Rep. 2021;7:5647–5666. doi: 10.1016/j.egyr.2021.08.196. [ DOI ] [ Google Scholar ]
- 16. Biofuels & Greenhouse Gas Emissions Myths versus facts. 2022. http://www.eere.energy.gov accessed.
- 17. Basak P., Chowdhury S., Halder Nee Dey S., Chowdhury S.P. A literature review on integration of distributed energy resources in the perspective of control, protection and stability of microgrid. Renew. Sustain. Energy Rev. 2012;16:5545–5556. doi: 10.1016/J.RSER.2012.05.043. [ DOI ] [ Google Scholar ]
- 18. Atikah M.S.N., Azlina W.A.K.G.W., Taufiq-Yap Y.H., Mahmoud O., El-Shafay A.S., Ilyas R.A., Harun R. Gasification characteristics and kinetics of lipid-extracted Nannochloropsis gaditana. Process. 2022;10:1525. doi: 10.3390/PR10081525. [ DOI ] [ Google Scholar ]
- 19. Pittman J.K., Dean A.P., Osundeko O. The potential of sustainable algal biofuel production using wastewater resources. Bioresour. Technol. 2011;102:17–25. doi: 10.1016/J.BIORTECH.2010.06.035. [ DOI ] [ PubMed ] [ Google Scholar ]
- 20. Simanjuntak J.P., Al-attab K.A., Daryanto E., Tambunan B.H. Eswanto, bioenergy as an alternative energy source: progress and development to meet the energy mix in Indonesia. J. Adv. Res. Fluid Mech. Therm. Sci. 2022;97:85–104. doi: 10.37934/ARFMTS.97.1.85104. [ DOI ] [ Google Scholar ]
- 21. Hannon M., Gimpel J., Tran M., Rasala B., Mayfield S. 2014. Biofuels from Algae: Challenges and Potential; pp. 763–784. [ DOI ] [ PMC free article ] [ PubMed ] [ Google Scholar ]
- 22. Sasikumar C., Sundaresan R., Nagaraja M., Rajaganapathy C. A review on energy generation from manure biomass. Mater. Today Proc. 2021;45:2408–2412. doi: 10.1016/J.MATPR.2020.10.832. [ DOI ] [ Google Scholar ]
- 23. Nazari A., Soltani M., Hosseinpour M., Alharbi W., Raahemifar K. Integrated anaerobic co-digestion of municipal organic waste to biogas using geothermal and CHP plants: a comprehensive analysis. Renew. Sustain. Energy Rev. 2021;152 doi: 10.1016/J.RSER.2021.111709. [ DOI ] [ Google Scholar ]
- 24. Yang Y., Tian Z., Lan Y., Wang S., Chen H. An overview of biofuel power generation on policies and finance environment, applied biofuels, device and performance. J. Traffic Transp. Eng. (English Ed. 2021;8:534–553. doi: 10.1016/J.JTTE.2021.07.002. [ DOI ] [ Google Scholar ]
- 25. Abanades S., Abbaspour H., Ahmadi A., Das B., Ehyaei M.A., Esmaeilion F., El Haj Assad M., Hajilounezhad T., Hmida A., Rosen M.A., Safari S., Shabi M.A., Silveira J.L. A conceptual review of sustainable electrical power generation from biogas. Energy Sci. Eng. 2022;10:630–655. doi: 10.1002/ESE3.1030. [ DOI ] [ Google Scholar ]
- 26. Olivares D.E., Mehrizi-Sani A., Etemadi A.H., Cañizares C.A., Iravani R., Kazerani M., Hajimiragha A.H., Gomis-Bellmunt O., Saeedifard M., Palma-Behnke R., Jiménez-Estévez G.A., Hatziargyriou N.D. Trends in microgrid control. IEEE Trans. Smart Grid. 2014;5:1905–1919. doi: 10.1109/TSG.2013.2295514. [ DOI ] [ Google Scholar ]
- 27. Ton D.T., Smith M.A. The U.S. Department of energy's microgrid initiative. Electr. J. 2012;25:84–94. doi: 10.1016/J.TEJ.2012.09.013. [ DOI ] [ Google Scholar ]
- 28. Boyce M.P. Handb. Cogener. Comb. Cycle Power Plants. second ed. 2010. Handbook for cogeneration and combined cycle power plants. [ DOI ] [ Google Scholar ]
- 29. Gu W., Wu Z., Yuan X. Microgrid economic optimal operation of the combined heat and power system with renewable energy. IEEE PES Gen. Meet. PES 2010. 2010 doi: 10.1109/PES.2010.5590140. [ DOI ] [ Google Scholar ]
- 30. Parhizi S., Lotfi H., Khodaei A., Bahramirad S. State of the art in research on microgrids: a review. IEEE Access. 2015;3:890–925. doi: 10.1109/ACCESS.2015.2443119. [ DOI ] [ Google Scholar ]
- 31. Kiehbadroudinezhad M., Merabet A., Hosseinzadeh-Bandbafha H. 2022. The Role of the Solar-Based Stand-Alone Microgrid to Enhance Environmental Sustainability: A Case Study. [ Google Scholar ]
- 32. Akorede M.F., Hizam H., Pouresmaeil E. Distributed energy resources and benefits to the environment. Renew. Sustain. Energy Rev. 2010;14:724–734. doi: 10.1016/J.RSER.2009.10.025. [ DOI ] [ Google Scholar ]
- 33. Pushparaj I., Karuthedath Parameswaran A., Srinivasan S.S., Kiehbadroudinezhad M., Merabet A., Hosseinzadeh-Bandbafha H. Review of latest advances and prospects of energy storage systems: considering economic, reliability, sizing, and environmental impacts approach. Cleanroom Technol. 2022;4:477–501. doi: 10.3390/CLEANTECHNOL4020029. 477–501. [ DOI ] [ Google Scholar ]
- 34. El-Khattam W., Salama M.M.A. Distributed generation technologies, definitions and benefits. Elec. Power Syst. Res. 2004;71:119–128. doi: 10.1016/J.EPSR.2004.01.006. [ DOI ] [ Google Scholar ]
- 35. Díaz-González F., Sumper A., Gomis-Bellmunt O., Villafáfila-Robles R. A review of energy storage technologies for wind power applications. Renew. Sustain. Energy Rev. 2012;16:2154–2171. doi: 10.1016/J.RSER.2012.01.029. [ DOI ] [ Google Scholar ]
- 36. Hosseinzadeh-Bandbafha H., Kiehbadroudinezhad M. Environmental impacts of chocolate production and consumption. Trends Sustain. Choc. Prod. 2022:229–258. doi: 10.1007/978-3-030-90169-1_7. [ DOI ] [ Google Scholar ]
- 37. Mariam L., Basu M., Conlon M.F. Microgrid: architecture, policy and future trends. Renew. Sustain. Energy Rev. 2016;64:477–489. doi: 10.1016/J.RSER.2016.06.037. [ DOI ] [ Google Scholar ]
- 38. Hosseinzadeh-Bandbafha H., Kiehbadroudinezhad M., Khanali M., Taghizadehghasab A. Emerging risks to plant health. Biodiversity, Funct. Ecosyst. Sustain. Food Prod. 2023:41–72. doi: 10.1007/978-3-031-07434-9_2. [ DOI ] [ Google Scholar ]
- 39. Kiehbadroudinezhad M., Rajabipour A., Cada M., Khanali M. Modeling, design, and optimization of a cost effective and reliable hybrid renewable energy system integrated with desalination using the division algorithm. Int. J. Energy Res. 2020;252 [ Google Scholar ]
- 40. Guerrero J.M., Loh P.C., Lee T.L., Chandorkar M. Advanced control architectures for intelligent microgridsPart II: power quality, energy storage, and AC/DC microgrids. IEEE Trans. Ind. Electron. 2013;60:1263–1270. doi: 10.1109/TIE.2012.2196889. [ DOI ] [ Google Scholar ]
- 41. Kiehbadroudinezhad M., Merabet A., Hosseinzadeh-Bandbafha H., Ghenai C. Environmental assessment of optimized renewable energy-based microgrids integrated desalination plant: considering human health, ecosystem quality, climate change, and resources. Environ. Sci. Pollut. Res. 2022;1:1–21. doi: 10.1007/S11356-022-24051-Z/FIGURES/8. [ DOI ] [ PubMed ] [ Google Scholar ]
- 42. Kiehbadroudinezhad S., Shahabi A., Kiehbadroudinezhad M.A. The spatial correlation of a multiple-input multiple-output and channel model using huygens-fresnel principle for underwater acoustic. J. Commun. Softw. Syst. 2019;15 doi: 10.24138/jcomss.v15i4.888. [ DOI ] [ Google Scholar ]
- 43. Kiehbadroudinezhad S., Bousquet J.-F., Cada M., Short C.I., Shahabi A., Kiehbadroudinezhad S., Kiehbadroudinezhad S., Bousquet J.-F., Cada M., Short C.I., Shahabi A., Kiehbadroudinezhad S. Expansion of a Y-shaped antenna array and optimization of the future antenna array in Malaysia for astronomical applications. J. Mod. Phys. 2019;10:888–908. doi: 10.4236/JMP.2019.108059. [ DOI ] [ Google Scholar ]
- 44. Majumder R. Some aspects of stability in microgrids. IEEE Trans. Power Syst. 2013;28:3243–3252. doi: 10.1109/TPWRS.2012.2234146. [ DOI ] [ Google Scholar ]
- 45. Zeng Z., Yang H., Zhao R. Study on small signal stability of microgrids: a review and a new approach. Renew. Sustain. Energy Rev. 2011;15:4818–4828. doi: 10.1016/J.RSER.2011.07.069. [ DOI ] [ Google Scholar ]
- 46. World rural population 1960-2022 | MacroTrends. 2022. https://www.macrotrends.net/countries/WLD/world/rural-population accessed.
- 47. Syafii Wati, Fahreza R. Techno-economic-enviro optimization analysis of diesel/pv/wind with pumped hydro storage for mentawai island microgrid. Bull. Electr. Eng. Informatics. 2021;10:2396–2404. doi: 10.11591/eei.v10i5.3167. [ DOI ] [ Google Scholar ]
- 48. Ortega-Arriaga P., Babacan O., Nelson J., Gambhir A. Grid versus off-grid electricity access options: a review on the economic and environmental impacts. Renew. Sustain. Energy Rev. 2021;143 doi: 10.1016/J.RSER.2021.110864. [ DOI ] [ Google Scholar ]
- 49. Coady J., Duquette J. Quantifying the impacts of biomass driven combined heat and power grids in northern rural and remote communities. Renew. Sustain. Energy Rev. 2021;148 doi: 10.1016/J.RSER.2021.111296. [ DOI ] [ Google Scholar ]
- 50. Li C., Zheng Y., Li Z., Zhang L., Zhang L., Shan Y., Tang Q. Techno-economic and environmental evaluation of grid-connected and off-grid hybrid intermittent power generation systems: a case study of a mild humid subtropical climate zone in China. Energy. 2021;230 doi: 10.1016/J.ENERGY.2021.120728. [ DOI ] [ Google Scholar ]
- 51. Shabani M., Dahlquist E., Wallin F., Yan J. Techno-economic impacts of battery performance models and control strategies on optimal design of a grid-connected PV system. Energy Convers. Manag. 2021;245 doi: 10.1016/j.enconman.2021.114617. [ DOI ] [ Google Scholar ]
- 52. Emad D., El-Hameed M.A., El-Fergany A.A. Optimal techno-economic design of hybrid PV/wind system comprising battery energy storage: case study for a remote area. Energy Convers. Manag. 2021;249 doi: 10.1016/J.ENCONMAN.2021.114847. [ DOI ] [ Google Scholar ]
- 53. Çetinbaş İ., Tamyürek B., Demirtaş M. Sizing optimization and design of an autonomous AC microgrid for commercial loads using Harris Hawks Optimization algorithm. Energy Convers. Manag. 2021;245 doi: 10.1016/J.ENCONMAN.2021.114562. [ DOI ] [ Google Scholar ]
- 54. Nafisi A., Arababadi R., Moazami A., Mahapatra K. Economic and emission analysis of running emergency generators in the presence of demand response programs. Energy. 2022;255 doi: 10.1016/J.ENERGY.2022.124529. [ DOI ] [ Google Scholar ]
- 55. de Oliveira Gabriel R., Leal Braga S., Pradelle F., Torres Serra E., Coutinho Sobral Vieira C.L. Numerical simulation of an on-grid natural gas PEMFC - solar photovoltaic micro CHP unit: analysis of the energy, economic and environmental impacts for residential and industrial applications. Technol. Econ. Smart Grids Sustain. Energy. 2022;7:1–19. doi: 10.1007/S40866-022-00124-3/TABLES/13. [ DOI ] [ Google Scholar ]
- 56. Jacal S., Straubinger F.B., Benjamin E.O., Buchenrieder G. Economic costs and environmental impacts of fossil fuel dependency in sub-Saharan Africa: a Nigerian dilemma. Energy Sustain. Dev. 2022;70:45–53. doi: 10.1016/J.ESD.2022.07.007. [ DOI ] [ Google Scholar ]
- 57. Nugroho R., Hanafi J., Shobatake K., Chun Y.-Y., Tahara K., Purwanto W.W. Life cycle inventories and life cycle assessment for an electricity grid network: case study of the Jamali grid, Indonesia. Int. J. Life Cycle Assess. 2022;27:1081–1091. doi: 10.1007/S11367-022-02082-5. [ DOI ] [ Google Scholar ]
- 58. Fares D., Fathi M., Mekhilef S. Performance evaluation of metaheuristic techniques for optimal sizing of a stand-alone hybrid PV/wind/battery system. Appl. Energy. 2022;305 doi: 10.1016/J.APENERGY.2021.117823. [ DOI ] [ Google Scholar ]
- 59. Makhloufi S., Khennas S., Bouchaib S., Arab A.H. Multi-objective cuckoo search algorithm for optimized pathways for 75% renewable electricity mix by 2050 in Algeria. Renew. Energy. 2022;185:1410–1424. [ Google Scholar ]
- 60. Singla M.K., Nijhawan P., Oberoi A.S. Cost–benefit comparison of fuel cell–based and battery-based renewable energy systems. Int. J. Energy Res. 2022;46:1736–1755. doi: 10.1002/ER.7291. [ DOI ] [ Google Scholar ]
- 61. Kiehbadroudinezhad M., Merabet A., Rajabipour A., Cada M., Kiehbadroudinezhad S., Khanali M., Hosseinzadeh-Bandbafha H. Optimization of wind/solar energy microgrid by division algorithm considering human health and environmental impacts for power-water cogeneration. Energy Convers. Manag. 2022;252 doi: 10.1016/J.ENCONMAN.2021.115064. [ DOI ] [ Google Scholar ]
- 62. Maheshwari P., Singla S., Shastri Y. Resiliency optimization of biomass to biofuel supply chain incorporating regional biomass pre-processing depots. Biomass Bioenergy. 2017;97:116–131. doi: 10.1016/J.BIOMBIOE.2016.12.015. [ DOI ] [ Google Scholar ]
- 63. Kiehbadroudinezhad M., Merabet A., Hosseinzadeh-Bandbafha H. A life cycle assessment perspective on biodiesel production from fish wastes for green microgrids in a circular bioeconomy. Bioresour. Technol. Reports. 2023;21 doi: 10.1016/J.BITEB.2022.101303. [ DOI ] [ Google Scholar ]
- 64. Shemfe M.B., Gu S., Ranganathan P. Techno-economic performance analysis of biofuel production and miniature electric power generation from biomass fast pyrolysis and bio-oil upgrading. Fuel. 2015;143:361–372. doi: 10.1016/J.FUEL.2014.11.078. [ DOI ] [ Google Scholar ]
- 65. World Energy Resources: 2013 Survey. World Energy Council; 2013. https://www.worldenergy.org/publications/entry/world-energy-resources-2013-survey (n.d.) accessed. [ Google Scholar ]
- 66. Fazendeiro L.M., Simões S.G. Historical variation of IEA energy and CO2 emission projections: implications for future energy modeling. Sustain. Times. 2021;13:7432. doi: 10.3390/SU13137432. [ DOI ] [ Google Scholar ]
- 67. Jabari F., Arasteh H., Sheikhi-Fini A., Ghaebi H., Bannae-Sharifian M.B., Mohammadi-Ivatloo B., Mohammadpourfard M. A biogas-steam combined cycle for sustainable development of industrial-scale water-power hybrid microgrids: design and optimal scheduling. Biofuels, Bioprod. Biorefining. 2022;16:172–192. doi: 10.1002/BBB.2300. [ DOI ] [ Google Scholar ]
- 68. Shepherd P. 2000. Biomass Co-firing: A Renewable Alternative for Utilities. [ Google Scholar ]
- 69. Schueftan A., González A.D. Reduction of firewood consumption by households in south-central Chile associated with energy efficiency programs. Energy Pol. 2013;63:823–832. doi: 10.1016/J.ENPOL.2013.08.097. [ DOI ] [ Google Scholar ]
- 70. Pristupa A.O., Mol A.P.J. Renewable energy in Russia: the take off in solid bioenergy? Renew. Sustain. Energy Rev. 2015;50:315–324. doi: 10.1016/J.RSER.2015.04.183. [ DOI ] [ Google Scholar ]
- 71. Bajwa D.S., Peterson T., Sharma N., Shojaeiarani J., Bajwa S.G. A review of densified solid biomass for energy production. Renew. Sustain. Energy Rev. 2018;96:296–305. doi: 10.1016/J.RSER.2018.07.040. [ DOI ] [ Google Scholar ]
- 72. Bojić S., Datkov D., Brcanov D., Georgijević M., Martinov M. Location allocation of solid biomass power plants: case study of Vojvodina. Renew. Sustain. Energy Rev. 2013;26:769–775. doi: 10.1016/J.RSER.2013.06.039. [ DOI ] [ Google Scholar ]
- 73. Guo M., Song W., Buhain J. Bioenergy and biofuels: history, status, and perspective. Renew. Sustain. Energy Rev. 2015;42:712–725. doi: 10.1016/J.RSER.2014.10.013. [ DOI ] [ Google Scholar ]
- 74. Library | biomass thermal energy council (BTEC) 2022. https://www.biomassthermal.org/resource/librarydfc6.html?kwSearch=Purchasing accessed.
- 75. Biomass magazine - the latest news on biomass power, fuels and chemical. 2022. https://biomassmagazine.com/plants/listplants/biomass/US/ accessed.
- 76. Biopower - Global Market Size Feedstock analysis, regulations and investment analysis to 2020. 2020. https://www.globaldata.com/store/report/biopower-global-market-size-feedstock-analysis-regulations-and-investment-analysis-to-2020/ accessed.
- 77. White M.S., Curtis M.L., Sarles R.L., Green D.W. Effects of outside storage on the energy potential of hardwood particulate fuels: Part II. Higher and net heating values. For. Prod. J. 1983;33:61–65. [ Google Scholar ]
- 78. Global Wood Pellet Industry - Market and Trade Study 2016 | Enhanced Reader. 2016. [ Google Scholar ]
- 79. Slaven I., Haviarova E., Cassens D. 2016. Properties of Wood Waste Stored for Energy Production. [ Google Scholar ]
- 80. Wood and Pellet Heating. Department of Energy; 2022. https://www.energy.gov/energysaver/wood-and-pellet-heating accessed. [ Google Scholar ]
- 81. de Meira A.M., Nolasco A.M., Klingenberg D., de Souza E.C., Dias Júnior A.F. Insights into the reuse of urban forestry wood waste for charcoal production. Clean Technol. Environ. Policy. 2021;23:2777–2787. doi: 10.1007/S10098-021-02181-1. [ DOI ] [ Google Scholar ]
- 82. Urbas J., Parker W.J. Surface temperature measurements on burning wood specimens in the Cone Calorimeter and the effect of grain orientation. Fire Mater. 1993;17:205–208. doi: 10.1002/FAM.810170502. [ DOI ] [ Google Scholar ]
- 83. Mensah K.E., Damnyag L., Kwabena N.S. 2020. Analysis of Charcoal Production with Recent Developments in Sub-sahara Africa: a Review. [ Google Scholar ]
- 84. Kajina W., Junpen A., Garivait S., Kamnoet O., Keeratiisariyakul P., Rousset P. 2020. Charcoal Production Processes: an Overview. [ Google Scholar ]
- 85. Nyamoga G.Z., Solberg B. A review of studies related to charcoal production, consumption, and greenhouse gas emissions in Tanzania. Clim. Chang. Manag. 2019:357–399. doi: 10.1007/978-3-030-12974-3_17/COVER. [ DOI ] [ Google Scholar ]
- 86. Chiong M.C., Chong C.T., Ng J.H., Lam S.S., Tran M.V., Chong W.W.F., Mohd Jaafar M.N., Valera-Medina A. Liquid biofuels production and emissions performance in gas turbines: a review. Energy Convers. Manag. 2018;173:640–658. doi: 10.1016/J.ENCONMAN.2018.07.082. [ DOI ] [ Google Scholar ]
- 87. Raud M., Kikas T., Sippula O., Shurpali N.J. Potentials and challenges in lignocellulosic biofuel production technology. Renew. Sustain. Energy Rev. 2019;111:44–56. doi: 10.1016/J.RSER.2019.05.020. [ DOI ] [ Google Scholar ]
- 88. del Río P.G., Gomes-Dias J.S., Rocha C.M.R., Romaní A., Garrote G., Domingues L. Recent trends on seaweed fractionation for liquid biofuels production. Bioresour. Technol. 2020;299 doi: 10.1016/J.BIORTECH.2019.122613. [ DOI ] [ PubMed ] [ Google Scholar ]
- 89. Devi A., Singh A., Bajar S., Owamah H.I. Nanomaterial in liquid biofuel production: applications and current status. Environ. Sustain. 2021;4:343–353. doi: 10.1007/S42398-021-00193-7. [ DOI ] [ Google Scholar ]
- 90. Singh S., Kumar A., Sivakumar N., Verma J.P. Deconstruction of lignocellulosic biomass for bioethanol production: recent advances and future prospects. Fuel. 2022;327 doi: 10.1016/J.FUEL.2022.125109. [ DOI ] [ Google Scholar ]
- 91. Prasad R.K., Chatterjee S., Mazumder P.B., Gupta S.K., Sharma S., Vairale M.G., Datta S., Dwivedi S.K., Gupta D.K. Bioethanol production from waste lignocelluloses: a review on microbial degradation potential. Chemosphere. 2019;231:588–606. doi: 10.1016/J.CHEMOSPHERE.2019.05.142. [ DOI ] [ PubMed ] [ Google Scholar ]
- 92. Naresh Kumar M., Ravikumar R., Thenmozhi S., Ranjith Kumar M., Kirupa Shankar M. Choice of pretreatment technology for sustainable production of bioethanol from lignocellulosic biomass: bottle necks and recommendations. Waste Biomass Valorization. 2018;10:1693–1709. [ Google Scholar ]
- 93. Badger P.C. 2002. Ethanol from Cellulose: A General Review. [ Google Scholar ]
- 94. EPA ignores reality with 2014 ethanol mandate - IER. 2014. https://www.instituteforenergyresearch.org/biofuel/epa-ignores-reality-with-2014-ethanol-mandate/ accessed.
- 95. Saravanan A., Senthil Kumar P., Jeevanantham S., Karishma S., Vo D.V.N. Recent advances and sustainable development of biofuels production from lignocellulosic biomass. Bioresour. Technol. 2022;344 doi: 10.1016/J.BIORTECH.2021.126203. [ DOI ] [ PubMed ] [ Google Scholar ]
- 96. Kaur T., Devi R., Kour D., Yadav N., Prasad S., Singh A., Negi P., Yadav A.N. 2020. Advances in Microbial Bioresources for Sustainable Biofuels Production: Current Research and Future Challenges; pp. 371–387. [ DOI ] [ Google Scholar ]
- 97. Pikula K., Zakharenko A., Stratidakis A., Razgonova M., Nosyrev A., Mezhuev Y., Tsatsakis A., Golokhvast K. 2020. The Advances and Limitations in Biodiesel Production: Feedstocks, Oil Extraction Methods, Production, and Environmental Life Cycle Assessment. http://mc.manuscriptcentral.com/tgcl [ Google Scholar ]
- 98. Moshood T.D., Nawanir G., Mahmud F. Microalgae biofuels production: a systematic review on socioeconomic prospects of microalgae biofuels and policy implications. Environ. Challenges. 2021;5 doi: 10.1016/J.ENVC.2021.100207. [ DOI ] [ Google Scholar ]
- 99. Kour D., Rana K.L., Yadav N., Yadav A.N., Rastegari A.A., Singh C., Negi P., Singh K., Saxena A.K. 2019. Technologies for Biofuel Production: Current Development, Challenges, and Future Prospects; pp. 1–50. [ DOI ] [ Google Scholar ]
- 100. Malode S.J., Prabhu K.K., Mascarenhas R.J., Shetti N.P., Aminabhavi T.M. Recent advances and viability in biofuel production. Energy Convers. Manag. X. 2021;10 doi: 10.1016/J.ECMX.2020.100070. [ DOI ] [ Google Scholar ]
- 101. Brahma S., Nath B., Basumatary B., Das B., Saikia P., Patir K., Basumatary S. Biodiesel production from mixed oils: a sustainable approach towards industrial biofuel production. Chem. Eng. J. Adv. 2022;10 doi: 10.1016/J.CEJA.2022.100284. [ DOI ] [ Google Scholar ]
- 102. Balachandran S.S. A comparative evaluation of usage of methyl esters of jatropha and fish oil for environmental protection. J. Mater. Environ. Sci. 2017;8:2564–2571. [ Google Scholar ]
- 103. Mattos C., Veloso M.C.C., Romeiro G.A., Folly E. Biocidal applications trends of bio-oils from pyrolysis: characterization of several conditions and biomass, a review. J. Anal. Appl. Pyrolysis. 2019;139:1–12. doi: 10.1016/J.JAAP.2018.12.029. [ DOI ] [ Google Scholar ]
- 104. Panchasara H., Ashwath N. Effects of pyrolysis bio-oils on fuel atomisation—a review. Energies. 2021;14:794. doi: 10.3390/EN14040794. [ DOI ] [ Google Scholar ]
- 105. Kaur R., Singh S.P. Commercial or pilot-scale pyrolysis units for conversion of biomass to bio-oils: state of the art, energy. Environ. Sustain. 2022:489–514. doi: 10.1007/978-981-16-8682-5_17/COVER. [ DOI ] [ Google Scholar ]
- 106. Staš M., Auersvald M., Kejla L., Vrtiška D., Kroufek J., Kubička D. Quantitative analysis of pyrolysis bio-oils: a review. TrAC, Trends Anal. Chem. 2020;126 doi: 10.1016/J.TRAC.2020.115857. [ DOI ] [ Google Scholar ]
- 107. Han X., Wang H., Zeng Y., Liu J. Advancing the application of bio-oils by co-processing with petroleum intermediates: a review. Energy Convers. Manag. X. 2021;10 doi: 10.1016/J.ECMX.2020.100069. [ DOI ] [ Google Scholar ]
- 108. Dell'Orco S., Rowland S.M., Harman-Ware A.E., Carpenter D., Foust T., Christensen E.D., Mukarakate C. 2021. Advanced Spectrometric Methods for Characterizing Bio-Oils to Enable Refineries to Reduce Fuel Carbon Intensity during Co-processing. [ Google Scholar ]
- 109. Gholizadeh M., Hu X., Liu Q. A mini review of the specialties of the bio-oils produced from pyrolysis of 20 different biomasses. Renew. Sustain. Energy Rev. 2019;114 doi: 10.1016/J.RSER.2019.109313. [ DOI ] [ Google Scholar ]
- 110. Mäki E., Saastamoinen H., Melin K., Matschegg D., Pihkola H. Drivers and barriers in retrofitting pulp and paper industry with bioenergy for more efficient production of liquid, solid and gaseous biofuels: a review. Biomass Bioenergy. 2021;148 doi: 10.1016/J.BIOMBIOE.2021.106036. [ DOI ] [ Google Scholar ]
- 111. Safieddin Ardebili S.M., Khademalrasoul A. An assessment of feasibility and potential of gaseous biofuel production from agricultural/animal wastes: a case study. Biomass Convers. Biorefinery. 2020:1–10. doi: 10.1007/S13399-020-00901-Z. [ DOI ] [ Google Scholar ]
- 112. Kapoor R., Ghosh P., Kumar M., Vijay V.K. Evaluation of biogas upgrading technologies and future perspectives: a review. Environ. Sci. Pollut. Res. 2019;26:11631–11661. doi: 10.1007/S11356-019-04767-1. [ DOI ] [ PubMed ] [ Google Scholar ]
- 113. Homepage U.S. Energy information administration (EIA) 2022. https://www.eia.gov/ accessed.
- 114. Kabeyi M.J.B., Olanrewaju O.A. Biogas production and applications in the sustainable energy transition. J. Energy. 2022;2022:1–43. [ Google Scholar ]
- 115. Yaqoob H., Teoh Y.H., Ud Din Z., Sabah N.U., Jamil M.A., Mujtaba M.A., Abid A. The potential of sustainable biogas production from biomass waste for power generation in Pakistan. J. Clean. Prod. 2021;307 doi: 10.1016/J.JCLEPRO.2021.127250. [ DOI ] [ Google Scholar ]
- 116. Anaerobic digestion of organic solid waste for energy production - satoto endar nayono - google books. 2022. https://books.google.ca/books?hl=en&lr=&id=cyZAAbGI6X0C&oi=fnd&pg=PR3&ots=MCwqREyw_Q&sig=xMtQTjQPNEpp4laM5NMOuYOc0xI&redir_esc=y#v=onepage&q&f=false accessed.
- 117. Chowdhury T., Chowdhury H., Hossain N., Ahmed A., Hossen M.S., Chowdhury P., Thirugnanasambandam M., Saidur R. Latest advancements on livestock waste management and biogas production: Bangladesh's perspective. J. Clean. Prod. 2020;272 doi: 10.1016/J.JCLEPRO.2020.122818. [ DOI ] [ Google Scholar ]
- 118. Lusk P. 1998. Methane Recovery from Animal Manures the Current Opportunities Casebook. [ DOI ] [ Google Scholar ]
- 119. Gotur N.M.R.B., Hemakumar Reddy R.M.P.G. 2022. Frequency Control of Renewables/Biodiesel/Biogas/Battery Energy Storage Based Isolated Microgrid Using the Honey Badger Algorithm; pp. 1–6. [ DOI ] [ Google Scholar ]
- 120. Kapoor R., Ghosh P., Kumar M., Sengupta S., Gupta A., Kumar S.S., Vijay V., Kumar V., Kumar Vijay V., Pant D. Valorization of agricultural waste for biogas based circular economy in India: a research outlook. Bioresour. Technol. 2020;304 doi: 10.1016/J.BIORTECH.2020.123036. [ DOI ] [ PubMed ] [ Google Scholar ]
- 121. Mishra A., Kumar M., Bolan N.S., Kapley A., Kumar R., Singh L. Multidimensional approaches of biogas production and up-gradation: opportunities and challenges. Bioresour. Technol. 2021;338 doi: 10.1016/J.BIORTECH.2021.125514. [ DOI ] [ PubMed ] [ Google Scholar ]
- 122. Zhao S., Bi X., Pan X., Su Y., Wu W. The optimization of in-situ tar reduction and syngas production on a 60-kW three-staged biomass gasification system: theoretical and practical approach. Biomass Convers. Biorefinery. 2019;11:1835–1846. [ Google Scholar ]
- 123. Abraham A., Mathew A.K., Park H., Choi O., Sindhu R., Parameswaran B., Pandey A., Park J.H., Sang B.I. Pretreatment strategies for enhanced biogas production from lignocellulosic biomass. Bioresour. Technol. 2020;301 doi: 10.1016/J.BIORTECH.2019.122725. [ DOI ] [ PubMed ] [ Google Scholar ]
- 124. Yan F., yi Luo S., quan Hu Z., Xiao B., Cheng G. Hydrogen-rich gas production by steam gasification of char from biomass fast pyrolysis in a fixed-bed reactor: influence of temperature and steam on hydrogen yield and syngas composition. Bioresour. Technol. 2010;101:5633–5637. doi: 10.1016/J.BIORTECH.2010.02.025. [ DOI ] [ PubMed ] [ Google Scholar ]
- 125. Tabatabaei M., Aghbashlo M., Valijanian E., Kazemi Shariat Panahi H., Nizami A.S., Ghanavati H., Sulaiman A., Mirmohamadsadeghi S., Karimi K. A comprehensive review on recent biological innovations to improve biogas production, Part 1: upstream strategies. Renew. Energy. 2020;146:1204–1220. doi: 10.1016/J.RENENE.2019.07.037. [ DOI ] [ Google Scholar ]
- 126. Wan C., Yu F., Zhang Y., Li Q., Wooten J. Material balance and energy balance analysis for syngas generation by a pilot-plant scale downdraft gasifier. J. Biobased Mater. Bioenergy. 2013;7:690–695. doi: 10.1166/JBMB.2013.1374. [ DOI ] [ Google Scholar ]
- 127. Sookramoon K. Updraft gasifier-stirling engine biomass incineration system power generation. Trends Sci. 2022;19:2170. doi: 10.48048/tis.2022.2170. [ DOI ] [ Google Scholar ]
- 128. Sapariya D.D., Patdiwala U.J., Panchal H., Ramana P.V., Makwana J., Sadasivuni K.K. 2021. A Review on Thermochemical Biomass Gasification Techniques for Bioenergy Production. [ DOI ] [ Google Scholar ]
- 129. Sikkema R., Proskurina S., Banja M., Vakkilainen E. How can solid biomass contribute to the EU's renewable energy targets in 2020, 2030 and what are the GHG drivers and safeguards in energy- and forestry sectors? Renew. Energy. 2021;165:758–772. doi: 10.1016/J.RENENE.2020.11.047. [ DOI ] [ Google Scholar ]
- 130. Mana A.A., Allouhi A., Ouazzani K., Jamil A. Feasibility of agriculture biomass power generation in Morocco: techno-economic analysis. J. Clean. Prod. 2021;295 doi: 10.1016/J.JCLEPRO.2021.126293. [ DOI ] [ Google Scholar ]
- 131. Moliner C., Marchelli F., Arato E. Current status of energy production from solid biomass in north-west Italy. Energies. 2020;13:4390. doi: 10.3390/EN13174390. [ DOI ] [ Google Scholar ]
- 132. Hartmann D., Kaltschmitt M. Electricity generation from solid biomass via co-combustion with coal: energy and emission balances from a German case study. Biomass Bioenergy. 1999;16:397–406. doi: 10.1016/S0961-9534(99)00017-3. [ DOI ] [ Google Scholar ]
- 133. Rajmohan K.S., Ramya C., Varjani S. 2019. Trends and Advances in Bioenergy Production and Sustainable Solid Waste Management. [ Google Scholar ]
- 134. Malico I., Nepomuceno Pereira R., Gonçalves A.C., Sousa A.M.O. Current status and future perspectives for energy production from solid biomass in the European industry. Renew. Sustain. Energy Rev. 2019;112:960–977. doi: 10.1016/J.RSER.2019.06.022. [ DOI ] [ Google Scholar ]
- 135. Hamzah N., Tokimatsu K., Yoshikawa K. Solid fuel from oil palm biomass residues and municipal solid waste by hydrothermal treatment for electrical power generation in Malaysia: a review. Sustain. Times. 2019;11:1060. doi: 10.3390/SU11041060. [ DOI ] [ Google Scholar ]
- 136. Purohit P., Chaturvedi V. Biomass pellets for power generation in India: a techno-economic evaluation. Environ. Sci. Pollut. Res. 2018;25:29614–29632. doi: 10.1007/S11356-018-2960-8/FIGURES/11. [ DOI ] [ PMC free article ] [ PubMed ] [ Google Scholar ]
- 137. Schneider T., Ruf F., Müller D., Karl J. Performance of a fluidized bed-fired Stirling engine as micro-scale combined heat and power system on wood pellets. Appl. Therm. Eng. 2021;189 doi: 10.1016/J.APPLTHERMALENG.2021.116712. [ DOI ] [ Google Scholar ]
- 138. Latif A., Das D.C., Biswas K., Kumar K., Kumar R., Hussain S.I. 2020. Non-critical Demands Managed Load Frequency Stabilization of Dish-Stirling-Biodiesel Based Islanded Microgrid System Using FF Optimized Controller; pp. 188–196. [ DOI ] [ Google Scholar ]
- 139. Bhuyan M., Das D.C., Barik A.K. Proficient power control strategy for combined solar gas turbine-wind turbine generator-biodiesel generator based two area interconnected microgrid employed with DC link using Harris's hawk optimization optimised tilt-integral-derivative controller. Int. J. Numer. Model. Electron. Network. Dev. Field. 2022;35 doi: 10.1002/JNM.2991. [ DOI ] [ Google Scholar ]
- 140. Feroldi D., Zumoffen D. Sizing methodology for hybrid systems based on multiple renewable power sources integrated to the energy management strategy. Int. J. Hydrogen Energy. 2014;39:8609–8620. doi: 10.1016/J.IJHYDENE.2014.01.003. [ DOI ] [ Google Scholar ]
- 141. Chiaramonti D., Oasmaa A., Solantausta Y. Power generation using fast pyrolysis liquids from biomass. Renew. Sustain. Energy Rev. 2007;11:1056–1086. doi: 10.1016/J.RSER.2005.07.008. [ DOI ] [ Google Scholar ]
- 142. Nagapurkar P., Smith J.D. Techno-economic optimization and social costs assessment of microgrid-conventional grid integration using genetic algorithm and artificial neural networks: a case study for two US cities. J. Clean. Prod. 2019;229:552–569. doi: 10.1016/J.JCLEPRO.2019.05.005. [ DOI ] [ Google Scholar ]
- 143. Keles S., Kaygusuz K. Fast pyrolysis of biomass for bio-oils. J. Eng. Res. Appl. Sci. 2016;5:370–377. http://www.journaleras.com/index.php/jeras/article/view/53 accessed. [ Google Scholar ]
- 144. Lak Kamari M., Maleki A., Alhuyi Nazari M., Sadeghi M., Rosen M.A., Pourfayaz F. Assessment of a biomass-based polygeneration plant for combined power, heat, bioethanol and biogas. Appl. Therm. Eng. 2021;198 doi: 10.1016/J.APPLTHERMALENG.2021.117425. [ DOI ] [ Google Scholar ]
- 145. Algieri A., Morrone P., Perrone D., Bova S., Castiglione T. Analysis of multi-source energy system for small-scale domestic applications. Integration of biodiesel, solar and wind energy. Energy Rep. 2020;6:652–659. doi: 10.1016/J.EGYR.2019.09.045. [ DOI ] [ Google Scholar ]
- 146. No S.Y. Application of bio-oils from lignocellulosic biomass to transportation, heat and power generation—a review. Renew. Sustain. Energy Rev. 2014;40:1108–1125. doi: 10.1016/J.RSER.2014.07.127. [ DOI ] [ Google Scholar ]
- 147. Bhuyan M., Barik A.K., Das D.C. 2020. GOA Optimised Frequency Control of Solar-Thermal/sea-Wave/biodiesel Generator Based Interconnected Hybrid Microgrids with DC Link. [ Google Scholar ]
- 148. Maleki A., Hajinezhad A., Rosen M.A. 2016. Modeling and Optimal Design of an Off-Grid Hybrid System for Electricity Generation Using Various Biodiesel Fuels: a Case Study for Davarzan. Iran. [ Google Scholar ]
- 149. Broumand M., Albert-Green S., Yun S., Hong Z., Thomson M.J. Spray combustion of fast pyrolysis bio-oils: applications, challenges, and potential solutions. Prog. Energy Combust. Sci. 2020;79 doi: 10.1016/J.PECS.2020.100834. [ DOI ] [ Google Scholar ]
- 150. Basmadjian N., Yun S., Hong Z. 2022. Optimization of Micro Gas Turbine Based Hybrid Systems for Remote Off-Grid Communities. [ DOI ] [ Google Scholar ]
- 151. Feroldi D., Degliuomini L.N., Basualdo M. Energy management of a hybrid system based on wind–solar power sources and bioethanol. Chem. Eng. Res. Des. 2013;91:1440–1455. doi: 10.1016/J.CHERD.2013.03.007. [ DOI ] [ Google Scholar ]
- 152. Armenta S., Robinson C., Bernal-Rubio K., Marin L.G., Jimenez-Estevez G., Mendoza P. Microgrid planning for isolated communities in Nuquí Colombia - IEEE CHILECON, 2021. IEEE Chil. Conf. Electr. Electron. Eng. Inf. Commun. Technol. CHILECON. 2021;2021 doi: 10.1109/CHILECON54041.2021.9703004. [ DOI ] [ Google Scholar ]
- 153. Tiwari S., Ongsakul W., Singh J.G. Design and simulation of an islanded hybrid microgrid for remote off-grid communities. Proc. 2020 Int. Conf. Util. Exhib. Energy, Environ. Clim. Chang. ICUE. 2020;2020 doi: 10.1109/ICUE49301.2020.9307028. [ DOI ] [ Google Scholar ]
- 154. Singh A., Basak P. Economic and environmental evaluation of rice straw processing technologies for energy generation: a case study of Punjab, India. J. Clean. Prod. 2019;212:343–352. doi: 10.1016/J.JCLEPRO.2018.12.033. [ DOI ] [ Google Scholar ]
- 155. Yıldız S., Gunduz H., Yildirim B., Temel Özdemir M. An islanded microgrid energy system with an innovative frequency controller integrating hydrogen-fuel cell. Fuel. 2022;326 doi: 10.1016/J.FUEL.2022.125005. [ DOI ] [ Google Scholar ]
- 156. Valenti F., Porto S.M.C., Dale B.E., Liao W. Spatial analysis of feedstock supply and logistics to establish regional biogas power generation: a case study in the region of Sicily. Renew. Sustain. Energy Rev. 2018;97:50–63. doi: 10.1016/J.RSER.2018.08.022. [ DOI ] [ Google Scholar ]
- 157. Kasaeian A., Rahdan P., Rad M.A.V., Yan W.M. Optimal design and technical analysis of a grid-connected hybrid photovoltaic/diesel/biogas under different economic conditions: a case study. Energy Convers. Manag. 2019;198 doi: 10.1016/J.ENCONMAN.2019.111810. [ DOI ] [ Google Scholar ]
- 158. Paulino R.F.S., Essiptchouk A.M., Silveira J.L. The use of syngas from biomedical waste plasma gasification systems for electricity production in internal combustion: thermodynamic and economic issues. Energy. 2020;199 doi: 10.1016/J.ENERGY.2020.117419. [ DOI ] [ Google Scholar ]
- 159. Xu J., Liu Z., Dai J. Environmental and economic trade-off-based approaches towards urban household waste and crop straw disposal for biogas power generation project -a case study from China. J. Clean. Prod. 2021;319 doi: 10.1016/J.JCLEPRO.2021.128620. [ DOI ] [ Google Scholar ]
- 160. Kohsri S., Meechai A., Prapainainar C., Narataruksa P., Hunpinyo P., Sin G. Design and preliminary operation of a hybrid syngas/solar PV/battery power system for off-grid applications: a case study in Thailand. Chem. Eng. Res. Des. 2018;131:346–361. doi: 10.1016/J.CHERD.2018.01.003. [ DOI ] [ Google Scholar ]
- 161. Abusoglu A., Tozlu A., Anvari-Moghaddam A. District heating and electricity production based on biogas produced from municipal WWTPs in Turkey: a comprehensive case study. Energy. 2021;223 doi: 10.1016/J.ENERGY.2021.119904. [ DOI ] [ Google Scholar ]
- 162. Nadaleti W.C. Utilization of residues from rice parboiling industries in southern Brazil for biogas and hydrogen-syngas generation: heat, electricity and energy planning. Renew. Energy. 2019;131:55–72. doi: 10.1016/J.RENENE.2018.07.014. [ DOI ] [ Google Scholar ]
- 163. Martín M., Grossmann I.E. Optimal integration of renewable based processes for fuels and power production: Spain case study. Appl. Energy. 2018;213:595–610. doi: 10.1016/J.APENERGY.2017.10.121. [ DOI ] [ Google Scholar ]
- 164. Gamarra Quintero J.S., Gonzalez C.A.D., Pacheco Sandoval L. Exergoeconomic analysis of a simulated system of biomass gasification-based power generation with surplus syngas storage in a rural zone in Colombia. Sustain. Energy Technol. Assessments. 2021;44 doi: 10.1016/J.SETA.2021.101075. [ DOI ] [ Google Scholar ]
- 165. Sigarchian S.G., Paleta R., Malmquist A., Pina A. Feasibility study of using a biogas engine as backup in a decentralized hybrid (PV/wind/battery) power generation system – case study Kenya. Energy. 2015;90:1830–1841. doi: 10.1016/J.ENERGY.2015.07.008. [ DOI ] [ Google Scholar ]
- 166. Prakash M., Sarkar A., Sarkar J., Mondal S.S., Chakraborty J.P. Proposal and design of a new biomass based syngas production system integrated with combined heat and power generation. Energy. 2017;133:986–997. doi: 10.1016/J.ENERGY.2017.05.161. [ DOI ] [ Google Scholar ]
- 167. Zhang C., Xu Y. Economic analysis of large-scale farm biogas power generation system considering environmental benefits based on LCA: a case study in China. J. Clean. Prod. 2020;258 doi: 10.1016/J.JCLEPRO.2020.120985. [ DOI ] [ Google Scholar ]
- 168. Jung S., Lee S., Park Y.K., Lee K.H., Kwon E.E. CO2-Mediated catalytic pyrolysis of rice straw for syngas production and power generation. Energy Convers. Manag. 2020;220 doi: 10.1016/J.ENCONMAN.2020.113057. [ DOI ] [ Google Scholar ]
- 169. Solarte-Toro J.C., Chacón-Pérez Y., Cardona-Alzate C.A. Evaluation of biogas and syngas as energy vectors for heat and power generation using lignocellulosic biomass as raw material. Electron. J. Biotechnol. 2018;33:52–62. doi: 10.1016/J.EJBT.2018.03.005. [ DOI ] [ Google Scholar ]
- 170. Sahoo B.P., Panda S. Load frequency control of solar photovoltaic/wind/biogas/biodiesel generator based isolated microgrid using harris hawks optimization. 1st Int. Conf. Power, Control Comput. Technol. ICPC2T. 2020;2020:188–193. doi: 10.1109/ICPC2T48082.2020.9071507. [ DOI ] [ Google Scholar ]
- 171. Beig B., Riaz M., Raza Naqvi S., Hassan M., Zheng Z., Karimi K., Pugazhendhi A., Atabani A.E., Thuy Lan Chi N. Current challenges and innovative developments in pretreatment of lignocellulosic residues for biofuel production: a review. Fuel. 2021;287 doi: 10.1016/J.FUEL.2020.119670. [ DOI ] [ Google Scholar ]
- 172. Nair S., Paulose H. Emergence of green business models: the case of algae biofuel for aviation. Energy Pol. 2014;65:175–184. doi: 10.1016/J.ENPOL.2013.10.034. [ DOI ] [ Google Scholar ]
- 173. Alalwan H.A., Alminshid A.H., Aljaafari H.A.S. Promising evolution of biofuel generations. Subject review. Renew. Energy Focus. 2019;28:127–139. doi: 10.1016/J.REF.2018.12.006. [ DOI ] [ Google Scholar ]
- 174. Li P., Sakuragi K., Makino H. Extraction techniques in sustainable biofuel production: a concise review. Fuel Process. Technol. 2019;193:295–303. doi: 10.1016/J.FUPROC.2019.05.009. [ DOI ] [ Google Scholar ]
- 175. Ganesan R., Manigandan S., Samuel M.S., Shanmuganathan R., Brindhadevi K., Lan Chi N.T., Duc P.A., Pugazhendhi A. A review on prospective production of biofuel from microalgae. Biotechnol. Reports. 2020;27 doi: 10.1016/J.BTRE.2020.E00509. [ DOI ] [ PMC free article ] [ PubMed ] [ Google Scholar ]
- 176. Abdullah B., ad Syed Muhammad S.A.F., Shokravi Z., Ismail S., Kassim K.A., Mahmood A.N., Aziz M.M.A. Fourth generation biofuel: a review on risks and mitigation strategies. Renew. Sustain. Energy Rev. 2019;107:37–50. doi: 10.1016/J.RSER.2019.02.018. [ DOI ] [ Google Scholar ]
- 177. Ambaye T.G., Vaccari M., Bonilla-Petriciolet A., Prasad S., van Hullebusch E.D., Rtimi S. Emerging technologies for biofuel production: a critical review on recent progress, challenges and perspectives. J. Environ. Manag. 2021;290 doi: 10.1016/J.JENVMAN.2021.112627. [ DOI ] [ PubMed ] [ Google Scholar ]
- 178. Correa D.F., Beyer H.L., Fargione J.E., Hill J.D., Possingham H.P., Thomas-Hall S.R., Schenk P.M. Towards the implementation of sustainable biofuel production systems. Renew. Sustain. Energy Rev. 2019;107:250–263. doi: 10.1016/J.RSER.2019.03.005. [ DOI ] [ Google Scholar ]
- 179. Suparmaniam U., Lam M.K., Uemura Y., Lim J.W., Lee K.T., Shuit S.H. Insights into the microalgae cultivation technology and harvesting process for biofuel production: a review. Renew. Sustain. Energy Rev. 2019;115 doi: 10.1016/J.RSER.2019.109361. [ DOI ] [ Google Scholar ]
- 180. Kumari P.A., Geethanjali P. Parameter estimation for photovoltaic system under normal and partial shading conditions: a survey. Renew. Sustain. Energy Rev. 2018;84:1–11. [ Google Scholar ]
- 181. Sharma S., Kundu A., Basu S., Shetti N.P., Aminabhavi T.M. Sustainable environmental management and related biofuel technologies. J. Environ. Manag. 2020;273 doi: 10.1016/J.JENVMAN.2020.111096. [ DOI ] [ PubMed ] [ Google Scholar ]
- 182. Long F., Liu W., Jiang X., Zhai Q., Cao X., Jiang J., Xu J. State-of-the-art technologies for biofuel production from triglycerides: a review. Renew. Sustain. Energy Rev. 2021;148 doi: 10.1016/J.RSER.2021.111269. [ DOI ] [ Google Scholar ]
Associated Data
This section collects any data citations, data availability statements, or supplementary materials included in this article.
Data Availability Statement
- View on publisher site
- PDF (6.4 MB)
- Collections
Similar articles
Cited by other articles, links to ncbi databases.
- Download .nbib .nbib
- Format: AMA APA MLA NLM
Add to Collections
- Biology & Environment
- Clean Energy
- Fusion & Fission
- Physical Sciences
- National Security
- Neutron Science
- Supercomputing
- User Facilities
- Educational Programs
- Procurement
- Small Business Programs
- Leadership Team
- Initiatives
- Visiting ORNL
- Fact Sheets
- Virtual Tour
Center for Bioenergy Innovation research supports Terragia biofuels approach
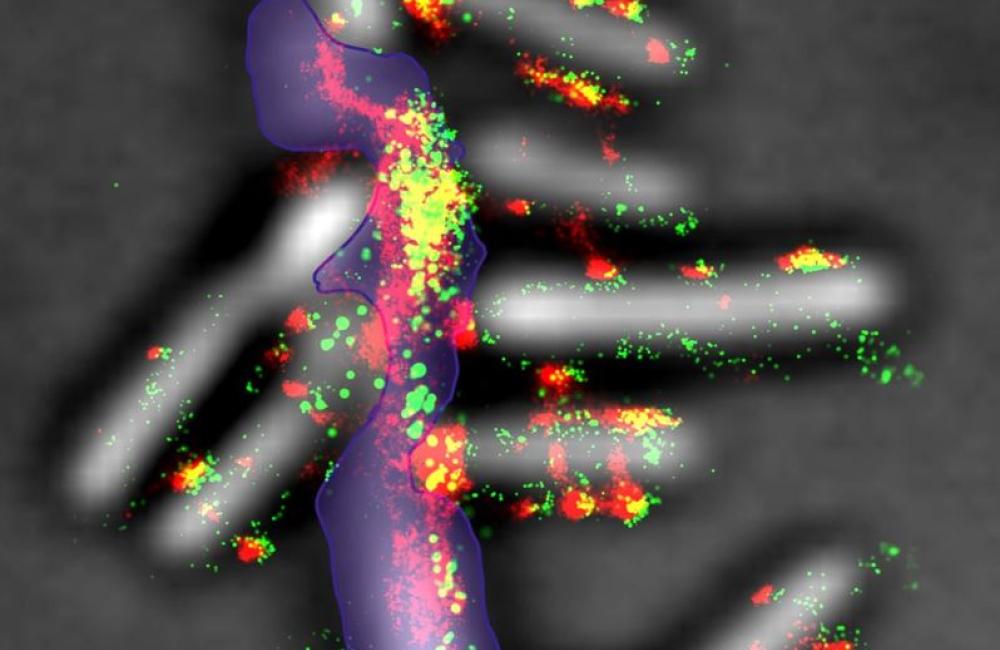
Clostridium thermocellum bacteria (gray/white) are deconstructing plant material (purple) in this image. The microbes digest and ferment plants simultaneously in a consolidated processing method to produce ethanol as a biofuel blendstock to power planes, ships and big rigs. Credit: National Renewable Energy Laboratory, U.S. Dept. of Energy
- Biology and Environment
Using technology developed by researchers working with the Center for Bioenergy Innovation at the Department of Energy’s Oak Ridge National Laboratory and Dartmouth College, startup company Terragia Biofuel is targeting commercial biofuels production that relies on plant waste material and consumes less energy. The technology can help meet the demand for billions of gallons of liquid biofuels for airplanes, ships and long-haul trucks.
New Hampshire-based Terragia plans to commercialize an approach that uses feedstocks such as plant-based lignocellulose — the tough, fibrous parts of plants that aren’t consumed as food — to make ethanol and other products at a much lower cost than today’s technology. The approach leverages bacteria that are good at digesting and converting plant waste in a one-step consolidated bioprocessing method without the addition of costly enzymes or thermochemical pretreatment currently used in conventional approaches to ethanol production.
The firm is advancing innovative approaches, including several licensed technologies resulting from work completed through the Center for Bioenergy Innovation , or CBI. CBI is a DOE Bioenergy Research Center that brings together about 250 scientists from 17 partner institutions, including Dartmouth and Terragia, to develop bioenergy-relevant plants, microbes and processing methods for biofuels production. CBI and its predecessor, the BioEnergy Science Center, or BESC, have generated nearly 40 patents for biofuels technologies that are available for licensing .
Terragia’s technology uses engineered bacteria such as Clostridium thermocellum that thrive at high temperatures and accomplish, in a single process, the release of soluble sugars from plant waste and fermentation of those sugars to make ethanol or other biofuels. The consolidated bioprocessing occurs without the addition of oxygen and has documented potential to substantially reduce capital costs and process energy inputs.
No-waste approach for jet fuel blendstocks
The method can utilize crop and forest residues as well as purpose-grown, nonfood perennial crops like poplar trees and switchgrass. The process yields “wet” ethanol, meaning it contains 20% water, that is then converted into blendstock fuels for jet engines. This microbial-driven process could be bolted onto existing ethanol refineries and use the corn stalks, cobs, husks and leaves typically treated as waste after the facilities process corn kernels.
“The ethanol produced by this consolidated bioprocessing method can be used in a certified jet fuel blendstock at a lower cost than what is currently being made in the corn-to-ethanol method because no further distillation is required of the intermediate wet ethanol,” said Gerald Tuskan, CBI director and ORNL Corporate Fellow. The method developed by CBI “allows current ethanol producers to take what is now a solid waste stream in their manufacturing process and convert it into additional ethanol output.”
The aviation industry has a goal of replacing 50% of its fuel, about 35 billion gallons, with sustainable options by the year 2050. The latest 2023 Billion-Ton report produced by ORNL for DOE’s Bioenergy Technologies Office revealed that the United States has more than 1 billion tons of nonfood plant-based feedstock available to produce such fuels.
“Terragia thinks of itself as a partner to industrial fuel producers,” said Terragia CEO Kristin Brief. “We envision working in close partnership with the firms who have access to plant feedstocks by supplying the technology to break down multiple types of plant material, enabling them to produce ethanol and other co-products.”
Terragia has raised $6 million in seed funding , led by investors Energy Impact Partners and The Engine, to help commercialize the biology-based technology. Terragia is co-locating its lab incubator space near CBI partner Dartmouth as it works on scaling the technology for industry. The company is currently working on a research and development project with a U.S. biofuels producer to adapt the consolidated bioprocessing method to a feedstock of interest.
“The biofuels industry understands that cellulosic plant-derived fuel is a missing link needed to reach aviation sector goals,” said Lee Lynd, a longtime collaborator with CBI/BESC, Distinguished Professor at Dartmouth and Chief Technology Officer at Terragia.
“CBI has made it possible for Terragia to bring the technology out into the world,” Lynd said.
Lynd referenced a large CBI study in 2022 that found producing cellulosic ethanol biologically and then catalytically upgrading it to larger hydrocarbon molecules for use in fuel blendstocks is a promising pathway for fuel production .
“With our years at CBI gaining fundamental understanding of the deconstruction process of lignocellulosic plant material, Terragia is now able to adopt that technology to create a business model that is attracting venture capital for further development into a commercial entity,” Tuskan said. CBI’s expertise dedicated to bioenergy research for a strong bioeconomy ranges from genomics and synthetic biology for plant and microbe development, to computational science, catalysis and chemical engineering and economics.
Lynd said CBI is “a shining example of an organization facilitating the kind of interdisciplinary research needed to move the science to the point where investors come in and propel a new company forward. CBI has kept its headlights on the road’s horizon rather than what’s just at its feet. Covering that distance has meant tackling work at a very large magnitude with dedication across a relatively long timeframe and sustaining a collaborative framework across multiple science areas.”
UT-Battelle manages ORNL for DOE’s Office of Science, the single largest supporter of basic research in the physical sciences in the United States. The Office of Science is working to address some of the most pressing challenges of our time. For more information, please visit energy.gov/science .

Media Contact
Researchers.

Organizations
Related news.
Biofuel beta
Fossil fuels are increasingly blended with biofuels—and getting the ratios just right requires technology developed by three national laboratories..
December 13, 2021
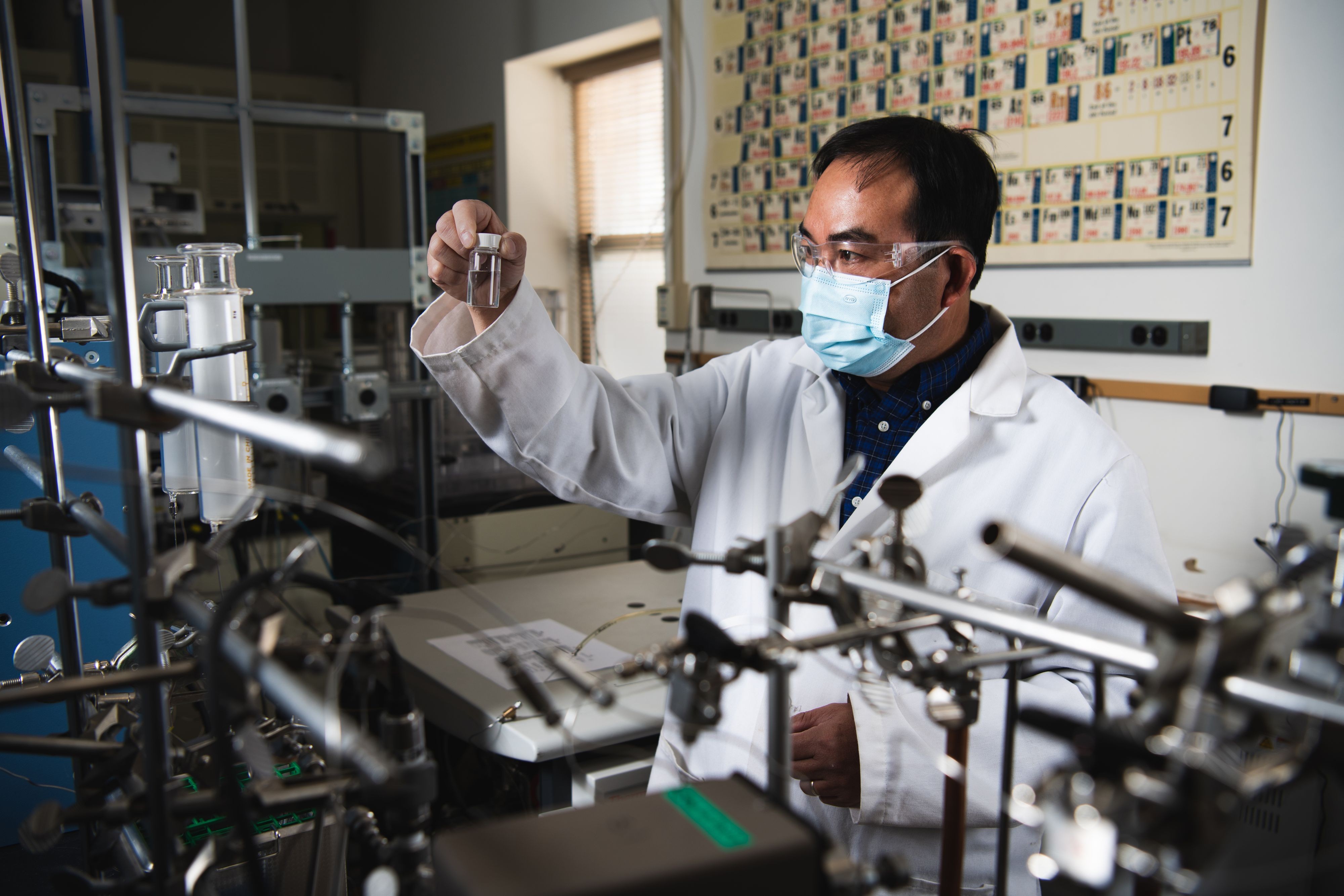
One day, all cars and trucks may run on electricity or hydrogen . But there are many years between now and that “one day.” To help the country reduce harmful greenhouse emissions in the interim, many companies are developing ways to supplement fossil fuels with cleaner biofuels.
For example, many companies mix fossil fuels, such as gasoline, with ethanol, a renewable carbon-based biofuel derived mostly from corn. Ethanol is considered an energy-positive fuel—meaning the amount of energy to produce it is less than the energy it produces. But like fossil fuels, biofuels undergo a complicated refining process, and this is where the Los Alamos National Laboratory has stepped in to help.
“It’s too expensive right now to build new refineries solely focused on biofuels,” says Zhenghua Li, of the Lab’s Earth System Observations group. “What we want to do is use existing refineries, and that requires understanding how much biofuel we can introduce into the co-processing system, and to do that we need to chemically track these biofuels.”
Li’s work focuses on chemically analyzing bio-fossil mixtures to ensure the percentage of renewable carbon fuel is what companies claim (so that they can receive a financial credit from the government), usually about 10 percent biofuel to 90 percent gasoline.
Often that biofuel is ethanol, but ethanol has a problem—as a derivative of corn or soy, it’s also a valuable food resource. So, the National Renewable Energy Lab in Golden, Colorado, and the Pacific Northwest National Laboratory in Richland, Washington, are developing biofuels from carbon sources that would otherwise go unused—everything from forest overgrowth to human fecal waste.
Scientists use pyrolysis, or extreme heat, to refine these waste sources into hydrocarbon-heavy oil, which can be sent to a refinery and co-processed. However, scientists are determining the ideal conditions for co-processing these types of waste, and they still need to know how much of their original mixture ends up in the final product.
One way to do this is by measuring carbon-13, an isotope of carbon. Li devised a way to trace how much carbon-13 a waste source begins with and how much of the isotope remains after the refining and co-processing. Li has also proposed an entirely new, much more efficient way for companies to track these metrics themselves. Normally, renewable carbon tests are conducted through an accelerator mass spectrometer, a device used for radiocarbon dating, which is expensive, limited, and time-consuming. Li, though, is working on tracing these carbon isotopes using a device that’s much more available, cheaper, and quicker—called an isotope ratio mass spectrometer.
Eventually, Li hopes these systems will be installed in refineries so that co-processed fuels can be characterized in real time.
More In This Issue
More national security science magazine stories.
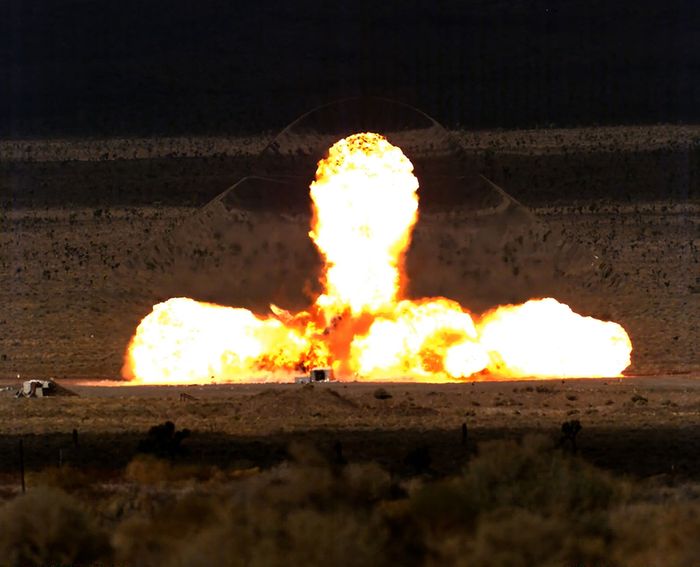
Photobomb NNSS
During a November 2020 experiment at the Nevada National Security Site, researchers detonated a 14-sided device that resulted in a spiked fireball and pyramidal shock wave.
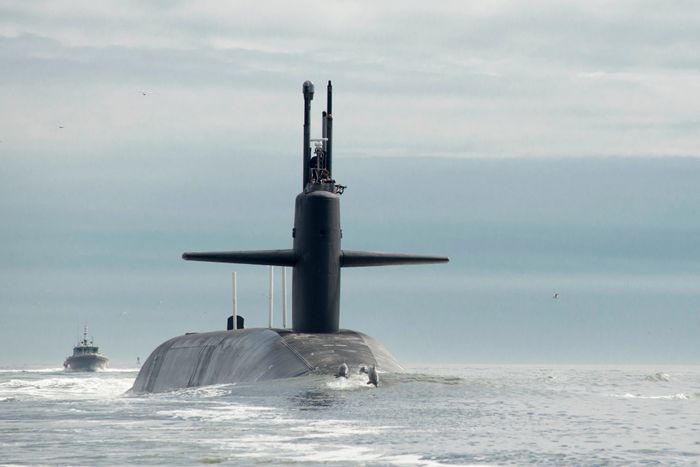
Major milestones for the W88
After a nearly 10-year update, the warhead is stockpile-bound.
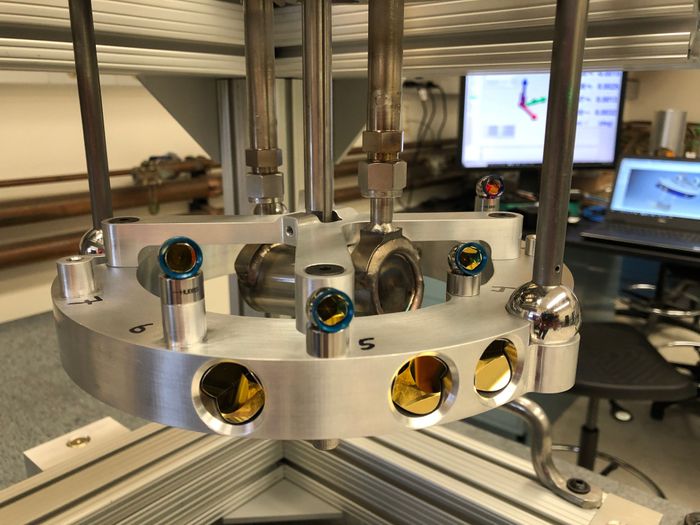
Better measurements for better physics
Upgrades at Weapons Neutron Research Facility enable new levels of precision.
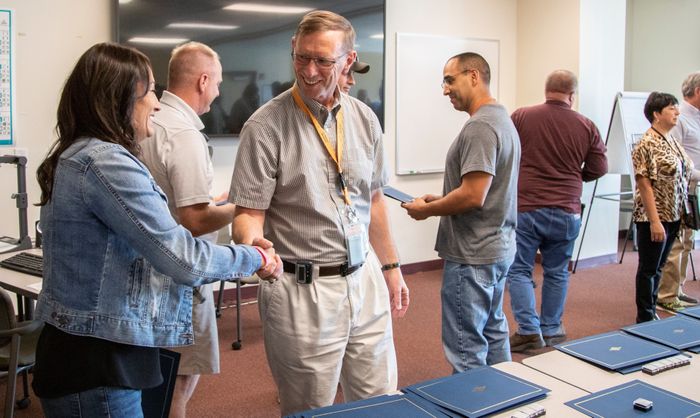
Ask an associate director: Dave Eyler
Dave Eyler, associate Laboratory director for Weapons Production, answers three questions.
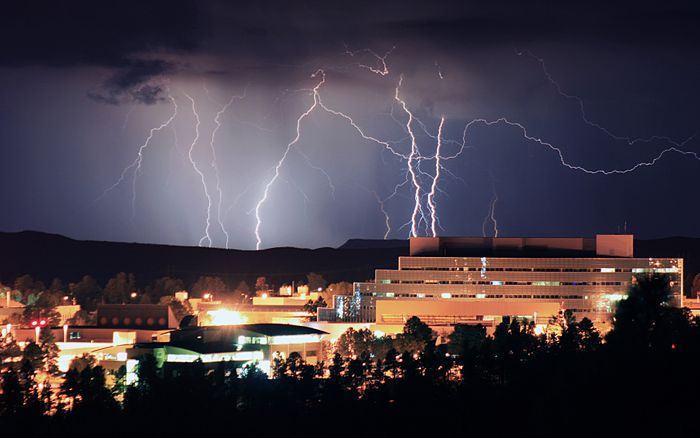
A laser focus on safety
Optical detonators will be less susceptible to accidental detonation.
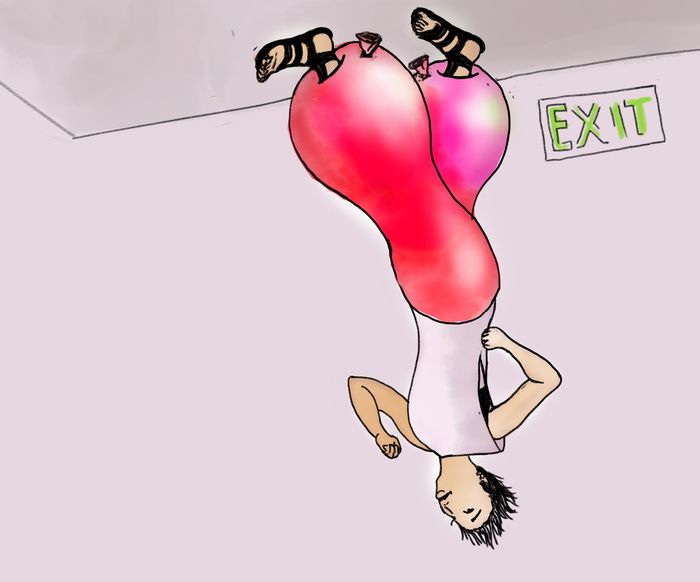
Building a better balloon
Scientists are on the cusp of solving an age-old engineering problem.

IMAGES
VIDEO
COMMENTS
Biofuels offer a solution to one of the challenges of solar, wind, and other alternative energy sources. These energy sources have incredible potential to reduce our dependence on fossil fuels and yield environmental and economic benefits. But many of these sources have a limitation: they can't replace liquid fuels such as jet fuel, gasoline ...
Ethanol (CH3CH2OH) is a renewable fuel that can be made from various plant materials, collectively known as " biomass.". Ethanol is an alcohol used as a blending agent with gasoline to increase octane and cut down carbon monoxide and other smog-causing emissions. The most common blend of ethanol is E10 (10% ethanol, 90% gasoline) and is ...
November 13, 2024. Office of Technology Transitions. Waste Into Energy: Biofuels Offer a Greener Future. Energy I-Corps equips teams from U.S. Department of Energy (DOE) National Labs with the tools to commercialize their technologies and bring them to market. The program pairs researchers with industry mentors and provides two months of ...
Biofuels could be a promising source to promote clean energy and resilience in microgrids; thus, they play a crucial role in the future development of the energy market. Biofuel helps reduce power generation industries' carbon footprint, recycle waste products, and have a superior energy balance.
Advantages. 1. Economic impact: biofuels cost similar to traditional fuels but have a smaller carbon footprint. In the long run, they tend to be more efficient with a reduced effect on the environment, with lesser emissions and fewer bioproducts they have an overall better economic value for a similar product [53] 2.
Using technology developed by researchers working with the Center for Bioenergy Innovation at the Department of Energy's Oak Ridge National Laboratory and Dartmouth College, startup company Terragia Biofuel is targeting commercial biofuels production that relies on plant waste material and consumes less energy. The technology can help meet ...
national security science. 2021 winter. biofuel beta. Biofuel beta. Fossil fuels are increasingly blended with biofuels—and getting the ratios just right requires technology developed by three national laboratories. December 13, 2021. Zhenghua Li conducts an experiment to quantify the renewable carbon content in a biofuel.
An accelerating global energy demand, paired with the harmful environmental effects of fossil fuels, has triggered the search for alternative, renewable energy sources. Biofuels are arguably a potential renewable energy source in the transportation industry as they can be used within current infrastructures and require less technological advances than other renewable alternatives, such as ...
In recent years, the increasing international energy demand and soaring oil prices have forced energy-consuming countries to turn their attention to alternative energy sources, especially the development of biofuels (Cabrera-Jiménez et al., 2022).Liquid biofuel production and consumption help combat climate change and promote agricultural diversity, energy security, and rural development ...
Summary. Rapid increases of energy consumption and human dependency on fossil fuels have led to the accumulation of greenhouse gases and consequently, climate change. As such, major efforts have been taken to develop, test, and adopt clean renewable fuel alternatives. Production of bioethanol and biodiesel from crops is well developed, while ...